The potential of nanomedicine therapies to treat neovascular disease in the retina
Journal of Angiogenesis Research. 2010;
Received: 25 June 2010 | Accepted: 8 October 2010 | Published: 8 October 2010
Vascular Cell ISSN: 2045-824X
Abstract
Neovascular disease in the retina is the leading cause of blindness in all age groups. Thus, there is a great need to develop effective therapeutic agents to inhibit and prevent neovascularization in the retina. Over the past decade, anti-VEGF therapeutic agents have entered the clinic for the treatment of neovascular retinal disease, and these agents have been effective for slowing and preventing the progression of neovascularization. However, the therapeutic benefits of anti-VEGF therapy can be diminished by the need for prolonged treatment regimens of repeated intravitreal injections, which can lead to complications such as endophthalmitis, retinal tears, and retinal detachment. Recent advances in nanoparticle-based drug delivery systems offer the opportunity to improve bioactivity and prolong bioavailability of drugs in the retina to reduce the risks associated with treating neovascular disease. This article reviews recent advances in the development of nanoparticle-based drug delivery systems which could be utilized to improve the treatment of neovascular disease in the retina.
Introduction
Retinopathy of prematurity (ROP), diabetic retinopathy (DR), and age-related macular degeneration (AMD), are the leading causes of blindness in infants, working-age adults, and the elderly, respectively [1–4]. These retinal diseases of varying etiology culminate with the development of pathogenic neovascularization, which disrupts retinal structure and function, causing irreversible vision loss. Although we understand much of the molecular mechanisms of neovascularization and have identified molecular targets and effective treatment options, sustaining safe and efficient drug delivery to the retina remains the primary obstacle to effectively treating neovascular disease in the retina. This is due to the inherent, isolated nature of the eye and the retina, which possesses a blood-retinal-barrier (BRB) to limit the diffusion of substances from the blood into the retina [5, 6].
The retina consists of seven layers of neuronal cells, including the photoreceptor cells which convert light stimuli into electrical signals that are sent through the other retinal neuronal cells to the optic nerve in order for visual perception to occur (Figure 1A). Adjacent to the photoreceptor cells, there is a monolayer of retinal pigment epithelial (RPE) cells. On the other side of the RPE cell monolayer, there is a basement membrane of extracellular matrix molecules known as Bruch's membrane, which separates the RPE from the choroidal vasculature. There are two levels of the BRB, the outer BRB (oBRB), which is formed by intercellular tight junctions in the RPE monolayer to restrict the passage of molecules from the choroidal blood supply into the neural retina, and the inner BRB (iBRB), which is formed by a monolayer of specialized non-fenestrated endothelial cells that form tight junctions within the retinal capillaries to prevent widespread diffusion of substances into the retina [5, 6]. The BRB is a major obstacle for drug delivery to treat retinal diseases [7]. Systemic drug dosing, via oral, intravenous, subcutaneous, or intraperitoneal administration is not very effective for drug delivery to the retina, since only 1-2% of the drug reaches the RPE and neural retina [8, 9]. Likewise, topical administration of drugs on the ocular surface in the form of eye drops or ointments is also inefficient for drug delivery to the retina. Thus, intravitreal (IVT) injection is most commonly used for drug administration to treat retinal disease. Although IVT injection can efficiently deliver drugs to the retina and RPE, prolonged treatment for chronic diseases often requires repeated injections, which can lead to severe complications, such as infections and retinal detachment.
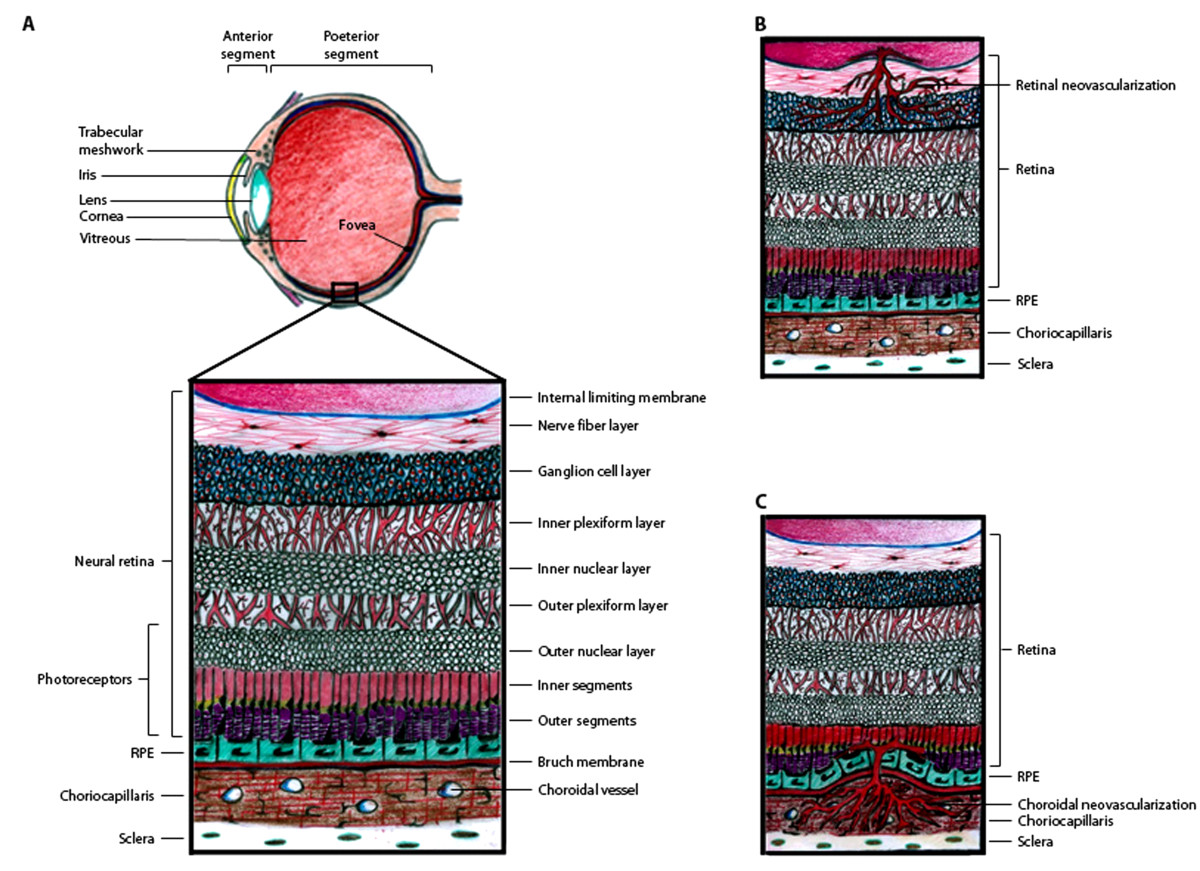
Figure 1
Figure 1 caption
Schematic representation of the retina and sites of pathogenic neovascularization. (A) Illustration of the eye, with the anterior segment consisting primarily of the lens, iris, and cornea and the posterior segment consisting primarily of the vitreous and retina. The small box highlights the location of the retinal tissue which lines the back of the eye and is diagramed in more detail. The retina is stratified into highly ordered layers as labeled in the picture. (B) Retinal neovascularization occurs when retinal capillaries pass through the inner limiting membrane and invade the retinal tissue, primarily in the ganglion cell layer. (C) Choroidal neovascularization occurs when choroidal capillaries pass through Bruch's membrane and invade the RPE and subretinal space.
DR and AMD are chronic, progressive diseases that lead to neovascularization within the retina. Therapeutic agents can slow and prevent the progression of neovascularization in DR and AMD, but the therapeutic benefits can be diminished by inefficient drug delivery and the limited duration of drug bioavailability, which requires prolonged treatment regimens of repeated IVT injections [10, 11]. Thus, improved drug delivery systems must be developed to treat neovascularization in DR and AMD. This article reviews the latest approaches to target and treat neovascular disease in the retina, with specific emphasis on recent preclinical studies in animal models and early-phase clinical trials aimed at developing nanomedicine modalities for more efficient and sustained delivery of therapeutic agents to the retina.
Cellular and Molecular Mechanisms of Pathogenic Neovascularization in the Retina
There are two types of neovascularization that occur in the retina and cause vision loss: retinal neovascularization (RNV) in which new vessels sprout from the retinal capillaries and invade the vitreous and neural retinal layers, and choroidal neovascularization (CNV) in which new vessels sprout from the choroidal vasculature and invade the subretinal space (Figure 1B and 1C). RNV can occur in both ROP and proliferative DR [1–3, 12], whereas CNV can occur in patients with AMD [13, 14]. Although RNV and CNV originate from different vascular networks and invade different layers of the retina, shared molecular mechanisms promote the progression of both.
In the pathogenesis of AMD, RPE cell function is impaired, which causes toxic cellular debris to accumulate intracellularly and underneath the basal surface of the RPE cell layer in the Bruch's membrane. Subsequently, RPE cell death can occur in patches known as geographic atrophy, and compromise the oBRB. At sites of geographic atrophy, ischemia and inflammation can promote CNV into the subretinal space. The newly forming blood vessels are leaky and cause inflammation and damage, resulting in photoreceptor cell death and permanent vision loss.
In DR, high blood glucose levels cause oxidative stress in endothelial cells, which results in cellular metabolic dysfunction and leads to retinal capillary basement membrane thickening. This initiates pericyte and endothelial cell death, resulting in breakdown of the iBRB. The loss of retinal capillary function causes vascular leakage and inflammation, as well as retinal ischemia, which promotes RNV and leads to irreversible vision loss.
ROP occurs in premature infants who are exposed to relative hyperoxia before the angiogenic phase of retinal development is complete [12]. This is problematic, since the angiogenic phase of retinal development is normally driven by hypoxia
Retinal ischemia is a common component of the pathogenesis of both CNV and RNV. Ischemia causes cellular hypoxia, which activates cellular signaling pathways to up-regulate the expression of angiogenic stimulators, such as vascular endothelial growth factor (VEGF) [15]. VEGF is a secreted glycoprotein with potent pro-angiogenic activity. VEGF binds to VEGF receptors (VEGFR) on endothelial cells to stimulate cell proliferation and migration. Numerous studies have shown that VEGF is up-regulated during the pathogenesis of CNV and RNV, and that VEGF is a key mediator of CNV and RNV pathogenesis [15].
Disrupted Balance of Angiogenic and Anti-Angiogenic Factors in RNV and CNV
The normal retina expresses a low amount of VEGF in the RPE, and high levels of angiogenic inhibitors, such as pigment epithelium-derived factor (PEDF) [16, 17]. PEDF is a secreted glycoprotein that belongs to the serine proteinase inhibitor (SERPIN) family, but does not have SERPIN activity. PEDF has potent anti-angiogenic activity and counteracts the effects of VEGF [18]. Thus, in the normal retinal homeostasis, the balance between pro- and anti-angiogenic factors tips in favor of angiogenic inhibition. This balance is disrupted during the pathogenesis of both CNV and RNV, as retinal ischemia promotes the up-regulation of VEGF expression and the down-regulation of PEDF expression, creating an increased VEGF/PEDF ratio that strongly promotes angiogenic stimulation during CNV and RNV [16, 17, 19].
Therapeutic interventions that either decrease the VEGF/PEDF ratio or inhibit VEGF activity can significantly inhibit CNV and RNV progression [11, 18, 20]. In rodent models, IVT injection of either recombinant PEDF protein or an adeno-associated viral plasmid expressing PEDF effectively decreases the VEGF/PEDF ratio and significantly reduces RNV and CNV [18, 21]. VEGF is the primary angiogenic stimulator in CNV and RNV, which has been highlighted by the clinical success of therapeutic agents that inhibit VEGF activity for the treatment of AMD and DR [11, 20]. However, anti-VEGF therapies have reduced efficacy during long-term treatment regimens. In a clinical study of patients with AMD, the efficacy of a single IVT injection of the anti-VEGF antibody Avastin® decreased to 50% of the initial dose response by the third IVT injection dose [22]. This phenomenon, known as tachyphylaxis, can contribute to the recurrence of neovascularization after anti-VEGF therapy.
Other angiogenic stimulators, such as platelet-derived growth factor (PDGF) and fibroblast growth factor (FGF) can also promote the pathogenesis of CNV and RNV, but therapeutically targeting either PDGF or FGF alone is not as effective as targeting VEGF activity; Nevertheless, studies suggest that combining PDGF or FGF inhibitors with VEGF inhibitors can have synergistic therapeutic effects in reducing the pathogenesis of CNV [23, 24]. In the future, combining therapies that target more than one angiogenic factor are likely to improve clinical outcome for AMD and DR patients,
In addition to PEDF, other angiogenic inhibitors are also expressed in the retina/RPE and have been implicated to play a role in the pathogenesis of CNV and RNV. For instance, another SERPIN family member, SERPINA3K, is an angiogenic inhibitor expressed in the normal retina that is down-regulated during the pathogenesis of RNV in DR [25]. In a rodent model of RNV, IVT injection of recombinant SERPINA3K protein decreased hypoxia-induced VEGF up-regulation and significantly reduced RNV and vascular leakage [26, 27]. Thrombopsondins (TSPs) are a type of secreted glycoprotein expressed by endothelial cells and RPE. TSP1 and TSP2 can inhibit endothelial cell proliferation and migration
Several angiogenic inhibitors are generated from the proteolytic cleavage products of native proteins, which display no angiogenesis-related activity prior to cleavage. One notable example is plasminogen, a pro-enzyme that is cleaved to generate the fibrinolytic enzyme plasmin. Additional cleavage of plasmin produces peptides with anti-angiogenic activity, including angiostatin and kringle 5 (K5). Angiostatin is a 38 kDa polypeptide which contains the first four triple disulfide bond-linked loops of plasminogen known as kringle domains [34]. Systemic (subcutaneous) or IVT injection of angiostatin reduces CNV, RNV and vascular leakage in rodent models [35–37]. K5 is the fifth kringle domain of plasminogen, consisting of only 80 amino acids. K5 is more potent than angiostatin for inhibiting bFGF-stimulated endothelial cell proliferation
Another group of angiogenic inhibitors, named vasoinhibins, are generated by the proteolytic cleavage of prolactin, growth hormone, or placental lactogen. Prolactin and prolactin-derived vasoinhibins are present in the retina [42], and prolactin-derived vasoinhibins can block VEGF-induced vasopermeability in rats with DR [43]. In rodent models, IVT injection of either antibodies against vasoinhibins or siRNA against prolactin causes retinal angiogenesis and vasodilation [42], whereas injection of recombinant vasoinhibin can suppress RNV [44]. These data suggest that prolactin-derived vasoinhibins are important angiogenic inhibitors in the retina.
Extracellular matrix (ECM) proteins, which are abundant in the retinal capillary basement membrane as well as the Bruch's membrane adjacent to the choriocapillaris, can also be cleaved to generate angiogenic inhibitors. The native or un-cleaved forms of these basement membrane proteins display no angiogenesis-related activity. This is intriguing, since the proteolytic digestion of the capillary basement membrane necessarily precedes angiogenic sprouting of new blood vessels. This implies that angiogenic inhibitors may be produced during early angiogenic sprouting in order to counterbalance angiogenic stimulators like VEGF and limit the extent of neovascularization. The most well-studied ECM-derived angiogenic inhibitor is endostatin, a 20 kDa C-terminal fragment derived from collagen XVIII alpha 1 (Col18α1) [45]. Endostatin is expressed in the human RPE [46], and its expression is decreased in AMD [19]. In a mouse model of laser-induced CNV,
Current Treatment Options for RNV and CNV
A common treatment for DR is laser-induced photocoagulation, in which a laser is used to alleviate hypoxia in the retina and attenuate RNV [52]. Although photocoagulation can stabilize vision and reduce the risk of future vision loss in many patients, there are significant risks associated with photocoagulation therapy, since the laser treatment alone can cause damage to the retina and permanently impair vision [52]. Furthermore, laser photocoagulation therapy does not stop the progression of DR in all patients. A similar, but safer laser-based method, photodynamic therapy (PDT), was the first FDA-approved therapy for the treatment of neovascular AMD. PDT utilizes a photo-activatable drug, verteporfin (Visudyne®, QLT Ophthalmics/Novartis AG), which is administered intravenously [53]. Vertoporfin collects in the choriocapillaris, and a low-energy laser beam is focused onto CNV lesions to activate verteporfin, which will induce blood clot formation to seal off abnormal neovascular blood vessels [53]. PDT cannot regress CNV lesions, but it can reduce the progression of CNV, although PDT must be repeated to sustain inhibition of vascular leakage [54].
A plethora of studies over the past decade have investigated the development of therapeutic agents that directly target the molecular mechanisms of angiogenesis. VEGF is the primary angiogenic stimulator in the pathogenesis of RNV and CNV [15]. Thus, several therapeutic agents have been designed to specifically inhibit VEGF activity, and such drugs have had clinical success in the treatment of DR and AMD [15]. In 2004, pegaptanib (Macugen®, Eyetech Inc.) was the first drug to obtain FDA approval for the treatment of CNV in AMD [55]. Macugen® is a 50 kDa RNA aptamer that binds to and inhibits VEGF [11, 55]. Also in 2004, a humanized monoclonal anti-VEGF antibody, bevacizumab (Avastin®, Genentech) was approved for anti-angiogenic therapy in cancer [56]. Avastin® is still in clinical trials for the treatment of AMD and DR, but it is routinely prescribed off-label for AMD patients [11, 56]. A smaller fragment of the bevacizumab antibody, ranibizumab (Lucentis®, Genentech) was FDA-approved specifically for the treatment of AMD in 2006, and is undergoing further clinical trials for the treatment of DR [11, 57]. Several clinical trials have shown that anti-VEGF therapeutic agents are more effective than PDT in maintaining and restoring visual acuity and reducing CNV progression in patients with AMD [10, 56]. Thus, other inhibitors of VEGF activity are also in development, including a soluble VEGFR mimetic, aflibercept (VEGF Trap-Eye™, Regeneron), and a siRNA that inhibits VEGF expression, bevasiranib (Cand5™, OPKO Health Inc.) [11]. The VEGF Trap-Eye™ is currently in Phase III clinical trials, and preliminary results have shown that it has been an effective treatment for CNV in AMD [58]. Clinical trials investigating the use of Cand5™ as a monotherapy were terminated in 2009 because Cand5™ therapy was less effective than Lucentis® therapy; however, Cand5™ is now in a clinical trial as a combination therapy administered in conjunction with Lucentis [11].
Although these anti-VEGF therapies have been effective for slowing disease progression and reducing the risk of vision loss due to AMD and DR, these therapies are limited by the need for burdensome and risky IVT injections, which must be repeated every 4-12 weeks in order to sustain therapeutic levels of the drugs in the retina [10, 11]. IVT injection can lead to vision-threatening complications, such as endophthalmitis, cataract, retinal tears, and retinal detachment [10, 59]. Thus, more effective drug delivery systems are desired to circumvent the need for IVT injection or at least reduce the frequency of IVT injections to thereby improve safety and increase patient compliance and patient outcome.
Developing Superior Therapeutic Agents with Nanotechnology
Nanotechnology offers the opportunity to create new drug delivery systems (DDS) to improve drug efficacy and safety for the treatment of neovascular disease in the retina. Nanotechnology has been defined as the design, characterization, production, and application of structures, devices, and systems by controlled manipulation of size and shape at the nanometer scale (atomic, molecular, and macromolecular scale) that produces structures, devices, and systems with at least one novel or superior characteristic or property [60]. Nanotechnology classically refers to matter in the size range of 1-100 nm, but it is often extended to include materials below 1 μm in size. The small size of nanotechnology materials could be especially useful for retinal drug delivery of systemically-administered drugs, which can be hindered by the BRB. Several studies have already demonstrated that some types of nanoparticles can cross the BRB to deliver therapeutics to the retina without exerting obvious cytotoxicity [61–63]. Furthermore, nanotechnology can be used to optimize drug formulations to increase drug solubility and alter pharmacokinetics to sustain drug release and thereby prolong bioavailability. In addition, the diverse platforms of nanotechnology can also be utilized to develop more sophisticated, cell-targeted therapies and to combine different drugs into one nanotherapeutic agent for synergistic therapeutic benefits.
Nanotechnology could be harnessed to reformulate anti-VEGF therapies for prolonged bioavailability and targeted delivery to neovascular lesions. However, nanotechnology-based DDS are in early stages of development, and reformulation of anti-VEGF therapies with nanotechnology-based DDS would require that new anti-VEGF "nanotherapies" be reevaluated for safety and efficacy in clinical trials, which is costly and time-consuming. Nevertheless, numerous preclinical studies suggest that nanotechnology-based DDS can address and overcome many of the challenges of retinal drug delivery to greatly improve therapeutic outcomes. This should encourage pharmaceutical scientists to co-develop nanotechnology-based DDS for new anti-neovascular therapeutic agents during preclinical development in order to generate superior nanotherapeutic agents for clinical trials.
Nanoparticle Platforms for Drug Delivery Systems
There is a diverse arsenal of nanoparticle systems available for the development of both simple and sophisticated nanotherapeutic agents to target neovascular disease in the retina. Nanoparticle platforms include synthetic and natural lipid-, polymer-, polypeptide-, and polysaccharide-based systems, as well as metallic nanoparticulates, such as gold [64–67]. Lipid-based nanoparticles can be used to generate liposomes, which consist of a phospholipid bilayer membrane that encapsulates cargo molecules [68]. Since naturally-occurring phospholipids are often used to generate liposomes, they are generally found to be biocompatible, non-toxic, and non-immunogenic. Liposomes can encapsulate either hydrophobic or hydrophilic molecules with high efficiency. Several liposome-based nanoparticle DDS have been FDA-approved for clinical use [68]. However, liposomes can be somewhat unstable, and stability can be improved by generating hybrid liposome-polymer nanoparticles. The polymeric compound polyethylene glycol (PEG) is most commonly used for this purpose. PEG is the most widely used polymeric nanoparticle system, and it can greatly extend the bioavailability of therapeutic agents.
The polymers polylactide (PLA) and polyglycolide (PGA) are also widely used for nanoparticle DDS. PLA and PGA are often mixed to generate the copolymer Poly(
In recent years, polymeric dendrimers have also been developed as nanoparticle DDS. Dendrimers are globular macromolecules which contain a central core element from which highly-branched structures emanate [73]. Dendrimer branches can be extended by stepwise synthesis, which allows for precise control of dendrimer structure, molecular weight, solubility, size, and shape. Thus, dendrimers are well-defined in size and composition compared to other nanoparticle DDS [73]. In addition, natural polymers, such as polypeptides and polysaccharides can also be used for nanoparticle DDS [67]. Polypeptide-based nanoparticles are most commonly generated using either albumin or poly-L-lysine, whereas polysaccharides, such as hyaluronic acid, heparin, chitosan, and cyclodextrin, can be formulated into nanoparticles alone or in combination with lipid-based or polymer-based nanoparticle platforms [64, 67, 74].
Metals, such as gold, silver, and platinum, can also be used for nanoparticle DDS. Gold is most commonly used, as it is inert, non-toxic, and non-immunogenic. A recent study showed that gold nanoparticles of 20 nm can pass through the BRB and exhibit no retinal toxicity, suggesting that gold nanoparticles could be used to safely and effectively deliver therapeutic agents to the retina [62]. Interestingly, naked gold nanoparticles have intrinsic anti-angiogenic activity. Moreover, gold nanoparticles conjugated with glycosaminoglycans have enhanced anti-angiogenic activity [75, 76]. This phenomenon has also been observed in chitosan nanoparticles and sixth generation poly-L-lysine dendrimers, which possess inherent anti-angiogenic activity [77, 78]. These observations warrant further investigation into the use of such nanoparticles for neovascular disease.
Development of Nanoparticle DDS to Treat Neovascular Disease in the Retina
Promising anti-neovascular therapeutic agents include gene therapy vectors, peptide-based inhibitors, antibodies, oligonucleotide aptamers, and small molecules. Some of these therapeutic agents have been combined with nanotechnology-based DDS in preclinical studies, resulting in increased and prolonged bioavailability, enhanced cell targeting, and overall increased therapeutic benefit compared to conventional DDS in animal models. The potential applications of nanoparticle-based DDS for the treatment of retinal neovascular disease are highlighted in the following sections.
Nanoparticles in Gene Therapy
Chronic and progressive retinal diseases, such as AMD and DR, require sustained delivery of therapeutic agents to the retina. As mentioned previously, although anti-angiogenic therapy with anti-VEGF agents has improved the treatment of AMD, these agents must be delivered to the retina by IVT injection every 4-12 weeks to maintain therapeutic benefits [10, 11]. Gene therapy-based delivery of anti-angiogenic factors could theoretically provide significantly prolonged therapeutic benefits after a single IVT injection.
The development of gene therapy vectors has surged over the past 15-20 years, and gene therapy has shown both significant successes and failures in the clinic [79, 80]. Viral vectors, such as recombinant adeno-associated viral vector (rAAV), have been most commonly used for gene therapy applications. However, there are significant safety concerns regarding the use of rAAV for gene therapy, as human clinical trials with rAAV have lead to oncogenesis and fatal systemic inflammation [79, 81–83]. In addition to the potential for adverse immunological responses, rAAV has a limited capacity for insert DNA (< 5 kb) as well as limited cell tropism [79]. Nevertheless, recent human clinical trials in patients with Leber's congenital amourosis caused by null mutations in RPE-specific protein 65 kDa (RPE65) have demonstrated that a single IVT injection of rAAV that expresses RPE65 can mediate expression of RPE65 for up to 1.5 years and improve vision without eliciting adverse immunological responses [80, 84, 85]; however, a transient increase in neutralizing antibodies to the rAAV capsid protein was observed [80]. Although the rAAV-RPE65 gene therapy results are hopeful at this point, the long-term safety and efficacy remains to be determined. rAAV-mediated gene therapy in the retina has been relatively safe thus far, due to the BRB-mediated immune-privileged state of the retina, although IVT injection of rAAV vectors in rats and dogs results in rAAV transfer to the brain [86, 87], suggesting that rAAV vectors should be used with caution.
As a potential treatment for CNV, a rAAV was generated to express recombinant human PEDF [21]. Periocular (scleral) injection of rAAV-PEDF resulted in increased PEDF expression in the retina, RPE, and choroid and resulted in a significant reduction in CNV lesions in mouse and pig models [21, 88]. In a recent Phase I clinical trial, rAAV-PEDF was administered by a single IVT injection to patients with neovascular AMD (CNV) [89]. The injection resulted in transient intraocular inflammation and increased intraocular pressure in 25% and 21% of patients, respectively. No other adverse inflammation occurred, suggesting the gene therapy was reasonably safe. Depending on the rAAV-PEDF dosage, between 50% and 71% of patients experienced either no change or improvement in CNV lesion size at 6 months post-injection. These results provide a proof-of-concept that angiogenic inhibitors can be delivered to the retina/RPE by gene therapy vectors; however, the use of non-viral vectors could reduce or prevent the incidence of intraocular inflammation observed with rAAV injection.
Non-viral DNA vectors offer a safe alternative to rAAV-mediated gene therapy, as non-viral vectors are non-immunogenic and non-toxic. Previously, the use of non-viral vectors has been limited due to low transfection efficiency and increased susceptibility to nuclease degradation. However, novel nanotechnology-based DDS have offered new potential for the use of non-viral vectors for gene therapy applications. Non-viral DNA vectors as large as 20 kb can now be compacted into nanoparticles of less than 25 nm in diameter, which allows the DNA to pass through nuclear pores [90]. This greatly enhances the transfection efficiency of non-viral vectors, especially in post-mitotic cells which could not be transfected by conventional non-viral DNA vectors [90–92]. Moreover, nanoparticle encapsulation also prolongs vector half-life by protecting the DNA from nuclease degradation.
In an effort to develop an efficient non-viral gene therapy vector for the treatment of RNV, we recently encapsulated a non-viral K5 expression plasmid into PLGA:Chitosan nanoparticles to produce a K5 nanoparticle expression vector (K5-NP) [93]. PLGA is a biocompatible, biodegradable polymer that is FDA-approved for use in humans [70]. PLGA nanoparticles have previously been shown to interact with the endo-lysosomal membrane and escape from the endocytic pathway into the cell cytosol, which may increase the delivery of PLGA nanoparticles to the nucleus [72]. Thus, PLGA-based nanoparticles are an attractive choice for gene therapy applications. The K5-NP was administered by IVT injection into rat models of ischemia-induced RNV and streptozotocin (STZ)-induced diabetes. We found that the K5-NP mediated expression of K5 in the retina for up to 4 weeks following a single IVT injection. The K5-NP expression was primarily restricted to the ganglion cell layer, with a high level of transfection efficiency. We demonstrated that the K5-NP significantly reduced ischemia-induced RNV, and decreased vascular leakage in both STZ-induced diabetes and ischemia-induced RNV [93]. The K5-NP prevented the up-regulation of VEGF and ICAM-1 in diabetic retinas for up to 4 weeks post-injection of the K5-NP. There was no detectable toxicity associated with the K5-NP, as histological analyses demonstrated that retinal structure and thickness was unaffected by K5-NP. Furthermore, the K5-NP did not increase retinal apoptotic cells, and electroretinography analyses showed that retinal physiology was normal following K5-NP injection. These studies demonstrate how nanoparticle-based DDS can facilitate non-viral gene therapy. Moreover, the K5-NP is an example of how gene therapy and nanotechnology can be combined to generate superior nanotherapeutics for the potential treatment of neovascular disease in the retina.
Peptide carriers can be incorporated into nanoparticles to enhance cellular uptake and avoid endolysosomal trafficking of cargo molecules, which may result in increased nuclear targeting of gene therapy vectors [94–96]. Peptide carriers include natural protein transduction domains and synthetic cell-penetrating peptides, which have the ability to traverse cell membranes without the use of transporters or cell surface receptors [94]. Natural protein transduction domains include the trans-activating regulatory protein (TAT) of human immunodeficiency virus and the VP22 protein from herpes simplex virus. Based on the molecular modeling of natural protein transduction domains, synthetic cell-penetrating peptides, such as Pep-1 and Pep-2 were developed. The Pep-1 and Pep-2 peptides consist of only 21 amino acid residues and contain 3 functionally distinct domains: a hydrophobic tryptophan-rich motif for cell membrane targeting, a hydrophilic lysine-rich domain derived from the SV40 large T antigen nuclear localization sequence which facilitates intracellular delivery, and a small linker domain which includes a proline residue to allow for flexibility [94]. Pep-1 and TAT peptides have been incorporated into nanoparticles to increase cellular and nuclear uptake of cargo molecules [97–100]. TAT-conjugation was able to increase nuclear targeting of 5 nm, but not 30 nm gold nanoparticles, suggesting that TAT-mediated trafficking to the nuclear compartment is restricted by nuclear pore dimensions [97–99].
Recently, a novel nanoparticle formulation was developed which compacts DNA to generate nanoparticles which contain a single DNA plasmid [92]. These nanoparticles utilize a 30-mer polylysine peptide which terminates with a single cysteine moiety (CK30). The terminal cysteine residue facilitates covalent bond formation with 10 kDa PEG to generate PEGylated CK30 (CK30-PEG). Plasmid DNA is then mixed with CK30-PEG to generate nanoparticles, and the size and shape of the nanoparticles can be adjusted by using different lysine amine counterions. Importantly, the minor diameter of each nanoparticle can be restricted to less than 25 nm, which allows CK30-PEG nanoparticles to traffic through nuclear pores [91, 101]. This likely explains how CK30-PEG DNA nanoparticles can mediate efficient gene expression in post-mitotic cell types [91, 92, 102]. The cellular uptake and nuclear targeting of CK30-PEG nanoparticles does not involve the endocytic pathway, but appears to be mediated at least in part by binding to nucleolin. Nucleolin is selectively expressed on the plasma membrane of specific cell types, including post-mitotic retinal cells [101, 103].
To investigate the potential use of CK30-PEG nanoparticles in retinal gene therapy, a reporter DNA plasmid which expressed green fluorescent protein (GFP) under the control of the cytomegalovirus promoter was compacted into CK30-PEG nanoparticles, and administered by IVT or subretinal (SRT) injection in mice [91]. SRT injection of CK30-PEG-GFP nanoparticles produced significant GFP expression in the RPE and retina, whereas IVT injection yielded significant GFP expression in the retina. Electroretinography analyses detected no abnormalities in retinal physiology due to the CK30-PEG-DNA nanoparticle injections. Total GFP expression in the retina was dependent on the amount of CK30-PEG-DNA nanoparticles injected. More recently, CK30-PEG nanoparticles were used to deliver a DNA plasmid which expressed the gene peripherin 2 (Prph2) to the retina of
Nanoparticles in Peptide and Drug Delivery
Therapeutic agents, including peptides, small molecule drugs, antibodies, and aptamers, can be formulated into nanoparticle-based DDS to improve therapeutic efficiency by increasing and prolonging bioavailability. The most simple nanotherapeutic agents are generated by condensing a therapeutic agent into nanoparticles using PEG or lipids. Thus, Macugen® is considered as a nanotherapeutic, since it is formulated using PEGylation to condense the drug into nanoparticles for enhanced drug delivery. Nanoparticle-based DDS can be especially helpful for drug molecules which have limited solubility or significant cytotoxic effects, such as the small molecule drug TNP-470, an analog of fumagillin [107].
TNP-470 is a very potent and effective angiogenic inhibitor, and in early studies it was very effective as an anti-tumor agent in several types of animal tumor models [107–112]. In human clinical trials, TNP-470 appeared to be an effective therapy for Kaposi's sarcoma, non-small-cell lung cancer, renal carcinoma, and prostate tumors [107–112]; however, clinical trials were terminated when TNP-470 elicited neurotoxic effects, including short-term memory loss, seizures, dizziness, and decreased motor coordination. TNP-470 is so small that it could easily penetrate the blood-brain-barrier (BBB) to elicit these effects. Initial attempts to reformulate TNP-470 to block penetration of the BBB resulted in a drug formulation with very transient bioavailability [113]. Recently, a nanotechnology-based DDS was developed for TNP-470 in which TNP-470 is conjugated to a di-block copolymer of monomethoxy-PEG-PLA, which self-assembles into nanomicilles of approximately 20 nm diameter [114]. This new formulation, named Lodamin, can be orally administered to effectively treat melanoma and lung cancer in animal models, with no evidence of BBB penetration or neurotoxicity. An ongoing preclinical study is evaluating the effects of Lodamin in a laser-induced CNV mouse model [115]. Lodamin was administered either by a daily oral dose of 15 mg/kg body weight, or as a single IVT injection of 100 μg or 300 μg. Therapeutic outcome was assessed at 14 days post-IVT injection or on the fourteenth consecutive day of daily oral treatment. Oral dosing was nearly as effective as a single IVT injection, as both oral dosing and IVT injection resulted in significantly reduced VEGF levels and a 70- 75% regression of CNV lesion size [115]. Thus, Lodamin is an example of how a small molecule anti-angiogenic drug can be reformulated with very simple nanotechnology-based DDS to alter drug pharmacokinetics and thereby greatly enhance therapeutic benefits and reduce toxic side effects.
A PLGA nanoparticle formulation of PEDF peptide was recently evaluated as a therapeutic agent in a mouse model of retinal ischemia [116]. The PLGA-PEDF nanoparticles were directly compared to treatment with PEDF peptide alone. Retinal ischemia rapidly induces retinal ganglion cell (RGC) death, and leads to thinning of the retina as apoptosis occurs in other retinal cell layers. IVT injection of either PLGA-PEDF nanoparticles or PEDF peptide alone significantly reduced RGC cell death; however, the PLGA-PEDF nanoparticles were significantly more effective. Furthermore, the PLGA-PEDF nanoparticles provided enhanced protection against RGC apoptosis for up to 7 days post-injection, whereas the PEDF peptide alone was only effective for up to 2 days. This study highlights how nanoparticle formulations can enhance and prolong the efficacy of a peptide-based drug. Furthermore, this suggests that a PLGA-PEDF peptide nanoparticle formulation could be therapeutically effective in treating retinal neovascular disease.
Nanoparticles for Targeted Drug Delivery
Nanoparticle carriers can greatly increase cell tropism and cell transfection efficiency; however, this can increase the non-specific uptake by non-target cells, including engulfing macrophages, which may result in decreased drug delivery to the target cell populations and increased drug side effects. Thus, modifying nanoparticles with cell-specific targeting agents can greatly enhance drug efficacy and reduce aberrant side effects. The nature of the nanoparticle formulation process allows for precise and stepwise synthesis of nanoparticle therapeutic agents. Nanoparticles that encapsulate a therapeutic agent can be constructed to carry various types of molecules on their external surface in order to target drug delivery to specific cell types. Moreover, more than one therapeutic agent can be combined into multi-layered nanoparticles to create a single nanotherapeutic agent which possesses synergistic therapeutic activity. Recent efforts to develop multi-component nanoparticle DDS which are specifically aimed at improving drug delivery to the retina and to neovascular retinal capillary endothelial cells are reviewed below.
Targeting Neovascular Endothelial Cells
Proliferating, neovascular endothelial cells up-regulate the expression of cell surface markers, such as intercellular adhesion molecule 1(ICAM1) and αvβ3 and αvβ5 integrins [117]. Antibodies or peptides designed to bind to these markers can be used to target drug delivery specifically to neovascular endothelial cells. The humanized monoclonal anti-αvβ3 integrin antibody known as etaracizumab (Abegrin®, MedImmune LLC) is already in clinical trials for cancer therapy, as it is expected to target tumor neovascularization [118, 119]. Extracellular matrix proteins which bind to integrins contain arginine-glycine-apartic acid (RGD) motifs. Synthetic cyclic and linear RGD peptides can bind to αvβ3 and αvβ5 integrins to mediate cellular uptake [117]. Various RGD peptides have been widely used in preclinical cancer studies to target tumor vasculature, and a cyclic RGD peptide which specifically binds both αvβ3 and αvβ5 integrins, Cilengitide (Merck) is in clinical trials for cancer therapy [120]. An anti-ICAM1 antibody has previously been conjugated to liposomes to generate immunoliposomes with enhanced endothelial cell uptake activity
A novel integrin-binding peptide (DFKLFAVYIKYR) known as C16Y, was derived from laminin-1, and functions independently as an integrin antagonist to inhibit angiogenesis [123]. In a laser-induced CNV rodent model, IVT injection of the C16Y peptide incorporated into PLA/polyethylene oxide(PEO) nanoparticles (PLA/PEO-C16YNP) was more effective than C16Y peptide alone for reducing CNV lesion size [124]. Moreover, the PLA/PEO-C16YNP had prolonged bioavailability compared to the C16Y peptide alone, demonstrating how nanoparticle formulations can enhance the bioactivity and bioavailability of therapeutic agents designed to target neovascular endothelial cells.
An ongoing preclinical study in mice utilizes quantum dot nanocrystals (QD) to generate ICAM1-targeted nanocarriers (ITNs) by conjugating ICAM1 antibodies to the external surface of the QD [125]. ITNs specifically target proliferating, neovascular endothelial cells, which selectively express ICAM1 on their cell surface. The ITNs, which are smaller than 200 nm, bind to ICAM-1 on the neovascular ECs, which leads to clathrin-mediated endocytosis of the ITNs. The ITNs can encapsulate various therapeutic agents, such as siRNA, peptides, and small molecules, and deliver those cargoes to the neovascular endothelial cells.
In addition to the use of nanocarriers as drug delivery agents, gold nanoparticles can also be used for photothermal-induced cell killing. Gold nanoparticles can be activated by a low-energy near-infrared laser to produce heat, which causes cell damage and death. This type of photothermal therapy has previously been explored for cancer treatment [126–128]. An ongoing preclinical study is investigating the use of gold nanoparticles for the photothermal treatment of CNV in AMD. In an effort to target neovascular endothelial cells in CNV lesions, PEG-coated gold nanorods of 45 nm × 15 nm were conjugated with RGD peptides (Gold-RGD-NP) [61]. Following intravenous administration in a CNV mouse model, Gold-RGD-NPs were localized in intracellular vesicles of retinal endothelial cells. Subsequently, laser treatment specifically induced cell death of endothelial cells containing Gold-RGD-NPs, whereas nearby cells which were not laser-treated and/or did not contain gold nanoparticles remained viable. The surrounding tissue is unharmed because the low-energy near-infrared laser does not generate heat unless it is focused onto the gold nanoparticles. Moreover, the heat that is generated by the gold nanoparticles is minimal and induces apoptosis, and not rapid necrosis, of neovascular endothelial cells. Although this study is in very early preclinical stages, it indicates that gold nanoparticle-mediated photothermal therapy could be a safe and effective treatment for CNV lesions in AMD and thus warrants follow-up studies. In future studies, gold nanorods could also be conjugated with different agents to target endothelial cells, such as antibodies which bind to the neovascular endothelial cell surface markers ICAM1 or αvβ3 integrin.
Enhancing Ocular Delivery
A recent study evaluated if nanoparticles, designed to target the retina and neovascular lesions, could be administered intravenously and result in effective gene delivery to CNV lesions [63]. This study utilized the Flt23K DNA plasmid, which encodes the anti-VEGF intraceptor, a recombinant protein that includes VEGF-binding domains 2 and 3 of VEGFR-1 coupled to the endoplasmic reticulum (ER) retention signal sequence Lys-Asp-Glu-Leu (KDEL) [129]. The anti-VEGF intraceptor is designed to bind to VEGF as it is synthesized in the ER to sequester VEGF and inhibit VEGF secretion. Previous studies have shown that the Flt23K plasmid can inhibit hypoxia-induced VEGF expression and corneal neovascularization
Preclinical studies have recently demonstrated that a synthetic cationic cell-penetrating peptide can facilitate the delivery of therapeutic agents, including peptides, small molecules, siRNA, and DNA, to the retina and RPE by IVT and SRT injection, respectively [95, 131]. This peptide for ocular delivery (POD), [CGGG(ARKKAAKA)4], was modified with PEG to generate nanoparticles which compact plasmid DNA into 120-150 nm nanoparticles [96]. Subretinal injection of PEG-POD-DNA nanoparticles resulted in DNA expression in RPE cells, and was 200-fold more efficient in transfecting RPE cells than naked DNA plasmid [96]. PEG-POD-DNA plasmid has since been used to deliver a neurotrophic factor to the mouse retina, which resulted in reduced light damage-induced retinal degeneration [132]. Thus, PEG-POD nanoparticles have the potential to be adapted for the delivery of anti-neovascular therapeutic agents to the retina and RPE for the treatment of RNV and CNV.
Conclusion
The treatment of retinal neovascular disease has been greatly improved by anti-VEGF therapies which have been developed over the past decade. However, frequent IVT injections are necessary for efficient and prolonged delivery of these therapeutic agents to the retina. Recent preclinical studies demonstrate that nanoparticle-based DDS can enhance bioactivity and prolong bioavailability of therapeutic agents in the retina. Moreover, efforts are underway to develop multi-component nanoparticle DDS to specifically target drug delivery to the retina, and more specifically to retinal neovascular endothelial cells. Thus, nanoparticle-based DDS are likely to have a large impact on the future treatment of neovascular disease in the retina.
Acknowledgements
The authors would like to thank Didier Nuno for designing and drawing the diagrams of the retina, RNV and CNV shown in Figure 1. The research cited in this work from our laboratory was supported by the National Eye Institute of the National Institutes of Health and a Center of Biomedical Research Excellence grant from the National Center for Research Resources.
Authors’ original submitted files for images
Below are the links to the authors’ original submitted files for images.
Authors’ original file for figure 1
References
- Diabetic retinopathy. N Engl J Med. 2004;350:48-58.
- Diabetic eye disease. Lancet. 1997;350:197-204.
- Incidence and early course of retinopathy of prematurity. The Cryotherapy for Retinopathy of Prematurity Cooperative Group. Ophthalmology. 1991;98:1628-1640.
- Global data on visual impairment in the year 2002. Bull World Health Organ. 2004;82:844-851.
- The blood-ocular barriers. Surv Ophthalmol. 1979;23:279-296.
- Blood-retinal barriers in health and disease. Trans Ophthalmol Soc U K. 1980;100:337-340.
- Recent perspectives in ocular drug delivery. Pharm Res. 2009;26:1197-1216.
- Drug delivery to the retina: challenges and opportunities. Expert Opin Biol Ther. 2003;3:45-56.
- Drug delivery for posterior segment eye disease. Invest Ophthalmol Vis Sci. 2000;41:961-964.
- Ranibizumab versus verteporfin photodynamic therapy for neovascular age-related macular degeneration: Two-year results of the ANCHOR study. Ophthalmology. 2009;116:57-65.
- Emerging pharmacologic therapies for wet age-related macular degeneration. Ophthalmologica. 2009;223:401-410.
- Sapieha P, Joyal JS, Rivera JC, Kermorvant-Duchemin E, Sennlaub F, Hardy P, Lachapelle P, Chemtob S: Retinopathy of prematurity: understanding ischemic retinal vasculopathies at an extreme of life. J Clin Invest. 120: 3022-3032. 10.1172/JCI42142.
- Age-related macular degeneration: etiology, pathogenesis, and therapeutic strategies. Surv Ophthalmol. 2003;48:257-293.
- Age-related macular degeneration (AMD): pathogenesis and therapy. Pharmacol Rep. 2006;58:353-363.
- Vascular endothelial growth factor in eye disease. Prog Retin Eye Res. 2008;27:331-371.
- Difference in ischemic regulation of vascular endothelial growth factor and pigment epithelium--derived factor in brown norway and sprague dawley rats contributing to different susceptibilities to retinal neovascularization. Diabetes. 2002;51:1218-1225.
- Unbalanced expression of VEGF and PEDF in ischemia-induced retinal neovascularization. FEBS Lett. 2001;489:270-276.
- Pigment epithelium-derived factor downregulates vascular endothelial growth factor (VEGF) expression and inhibits VEGF-VEGF receptor 2 binding in diabetic retinopathy. J Mol Endocrinol. 2006;37:1-12.
- Reduction of endogenous angiogenesis inhibitors in Bruch's membrane of the submacular region in eyes with age-related macular degeneration. Arch Ophthalmol. 2008;126:670-678.
- Anti-VEGF therapy in proliferative diabetic retinopathy. Int Ophthalmol Clin. 2009;49:95-107.
- Periocular injection of an adenoviral vector encoding pigment epithelium-derived factor inhibits choroidal neovascularization. Gene Ther. 2003;10:637-646.
- Is there tachyphylaxis to intravitreal anti-vascular endothelial growth factor pharmacotherapy in age-related macular degeneration?. Ophthalmology. 2008;115:2199-2205.
- Inhibition of platelet-derived growth factor B signaling enhances the efficacy of anti-vascular endothelial growth factor therapy in multiple models of ocular neovascularization. Am J Pathol. 2006;168:2036-2053.
- Combinatory inhibition of VEGF and FGF2 is superior to solitary VEGF inhibition in an in vitro model of RPE-induced angiogenesis. Graefes Arch Clin Exp Ophthalmol. 2009;247:767-773.
- Kallikrein-binding protein levels are reduced in the retinas of streptozotocin-induced diabetic rats. Invest Ophthalmol Vis Sci. 1997;38:658-664.
- Kallikrein-binding protein inhibits retinal neovascularization and decreases vascular leakage. Diabetologia. 2003;46:689-698.
- Anti-inflammatory and antioxidant effects of SERPINA3K in the retina. Invest Ophthalmol Vis Sci. 2009;50:3943-3952.
- Thrombospondin-1, PECAM-1, and regulation of angiogenesis. Histol Histopathol. 1999;14:285-294.
- Impaired expression of thrombospondin-1 in eyes with age related macular degeneration. Br J Ophthalmol. 2006;90:48-54.
- Thrombospondin-1-deficient mice exhibit increased vascular density during retinal vascular development and are less sensitive to hyperoxia-mediated vessel obliteration. Dev Dyn. 2003;228:630-642.
- Attenuation of retinal vascular development and neovascularization in transgenic mice over-expressing thrombospondin-1 in the lens. Dev Dyn. 2006;235:1908-1920.
- Role of hypoxia and extracellular matrix-integrin binding in the modulation of angiogenic growth factors secretion by retinal pigmented epithelial cells. J Cell Biochem. 1999;74:135-143.
- Attenuation of proliferation and migration of retinal pericytes in the absence of thrombospondin-1. Am J Physiol Cell Physiol. 2009;296:C724-734.
- Angiostatin: a novel angiogenesis inhibitor that mediates the suppression of metastases by a Lewis lung carcinoma. Cell. 1994;79:315-328.
- Angiostatin inhibits pathological but not physiological retinal angiogenesis. Invest Ophthalmol Vis Sci. 2001;42:3325-3330.
- Recombinant adeno-associated virus vector expressing angiostatin inhibits preretinal neovascularization in adult rats. Ophthalmic Res. 2005;37:50-56.
- Suppression of choroidal neovascularization by adeno-associated virus vector expressing angiostatin. Invest Ophthalmol Vis Sci. 2001;42:2401-2407.
- Kringle 5 of plasminogen is a novel inhibitor of endothelial cell growth. J Biol Chem. 1997;272:22924-22928.
- Down-regulation of vascular endothelial growth factor and up-regulation of pigment epithelium-derived factor: a possible mechanism for the anti-angiogenic activity of plasminogen kringle 5. J Biol Chem. 2002;277:9492-9497.
- Intravitreal injection of plasminogen kringle 5, an endogenous angiogenic inhibitor, arrests retinal neovascularization in rats. Diabetologia. 2001;44:757-765.
- Plasminogen kringle 5 reduces vascular leakage in the retina in rat models of oxygen-induced retinopathy and diabetes. Diabetologia. 2004;47:124-131.
- Prolactins are natural inhibitors of angiogenesis in the retina. Invest Ophthalmol Vis Sci. 2005;46:2947-2953.
- Vasoinhibins prevent retinal vasopermeability associated with diabetic retinopathy in rats via protein phosphatase 2A-dependent eNOS inactivation. J Clin Invest. 2008;118:2291-2300.
- Vasohibin is up-regulated by VEGF in the retina and suppresses VEGF receptor 2 and retinal neovascularization. Faseb J. 2006;20:723-725.
- Endostatin: an endogenous inhibitor of angiogenesis and tumor growth. Cell. 1997;88:277-285.
- Collagen XVIII/endostatin is essential for vision and retinal pigment epithelial function. Embo J. 2004;23:89-99.
- Endogenous endostatin inhibits choroidal neovascularization. Faseb J. 2007;21:3809-3818.
- Phase I clinical trial of recombinant human endostatin administered as a short intravenous infusion repeated daily. J Clin Oncol. 2002;20:3772-3784.
- Phase II study of recombinant human endostatin in patients with advanced neuroendocrine tumors. J Clin Oncol. 2006;24:3555-3561.
- [Randomized phase II trial on escalated doses of Rh-endostatin (YH-16) for advanced non-small cell lung cancer]. Zhonghua Zhong Liu Za Zhi. 2006;28:138-141.
- Extracellular matrix-derived peptide binds to alpha(v)beta(3) integrin and inhibits angiogenesis. J Biol Chem. 2001;276:31959-31968.
- Lasers and diabetic retinopathy: the art of gentle destruction. Diabetes Technol Ther. 1999;1:177-187.
- Photodynamic therapy of subfoveal choroidal neovascularization in age-related macular degeneration with verteporfin: two-year results of 2 randomized clinical trials-tap report 2. Arch Ophthalmol. 2001;119:198-207.
- Photodynamic therapy with verteporfin in age-related macular degeneration: a systematic review of efficacy, safety, treatment modifications and pharmacoeconomic properties. Acta Ophthalmol. 2009;87:118-132.
- Pegaptanib, a targeted anti-VEGF aptamer for ocular vascular disease. Nat Rev Drug Discov. 2006;5:123-132.
- Randomised clinical trial of intravitreal Avastin vs photodynamic therapy and intravitreal triamcinolone: long-term results. Eye (Lond). 2009;23:2223-2227.
- Randomized trial evaluating ranibizumab plus prompt or deferred laser or triamcinolone plus prompt laser for diabetic macular edema. Ophthalmology. 2010;117:1064-1077.
- A phase I study of intravitreal vascular endothelial growth factor trap-eye in patients with neovascular age-related macular degeneration. Ophthalmology. 2009;116:2141-2148.
- Complications of intravitreal injections. Curr Opin Ophthalmol. 2010;21:178-183.
- Protecting new ideas and inventions in nanomedicine with patents. Nanomedicine. 2005;1:150-158.
- Laser-Activated Gold-Nanoparticles as a Potential New Treatment Modality for Exudative Age-Related Macular Degeneration [abstract]. IOVS. 2010.
- Intravenously administered gold nanoparticles pass through the blood-retinal barrier depending on the particle size, and induce no retinal toxicity. Nanotechnology. 2009;20:505101-.
- Intravenous transferrin, RGD peptide and dual-targeted nanoparticles enhance anti-VEGF intraceptor gene delivery to laser-induced CNV. Gene Ther. 2009;16:645-659.
- Nanoparticles in medicine: therapeutic applications and developments. Clin Pharmacol Ther. 2008;83:761-769.
- Biological properties of "naked" metal nanoparticles. Adv Drug Deliv Rev. 2008;60:1289-1306.
- Gold nanoparticles in delivery applications. Adv Drug Deliv Rev. 2008;60:1307-1315.
- Nanoparticulate systems for growth factor delivery. Pharm Res. 2009;26:1561-1580.
- Recent advances with liposomes as pharmaceutical carriers. Nat Rev Drug Discov. 2005;4:145-160.
- Polymer degradation and in vitro release of a model protein from poly(D,L-lactide-co-glycolide) nano- and microparticles. J Control Release. 2003;92:173-187.
- Biodegradable nanoparticles for drug and gene delivery to cells and tissue. Adv Drug Deliv Rev. 2003;55:329-347.
- Polymer conjugates as anticancer nanomedicines. Nat Rev Cancer. 2006;6:688-701.
- Rapid endo-lysosomal escape of poly(DL-lactide-co-glycolide) nanoparticles: implications for drug and gene delivery. Faseb J. 2002;16:1217-1226.
- Dendrimers in gene delivery. Adv Drug Deliv Rev. 2005;57:2177-2202.
- Polysaccharides-based nanoparticles as drug delivery systems. Adv Drug Deliv Rev. 2008;60:1650-1662.
- Gold and silver nanoparticles conjugated with heparin derivative possess anti-angiogenesis properties. Nanotechnology. 2009;20:455104-.
- Synthesis of gold and silver nanoparticles stabilized with glycosaminoglycans having distinctive biological activities. Biomacromolecules. 2009;10:589-595.
- Systemic antiangiogenic activity of cationic poly-L-lysine dendrimer delays tumor growth. Proc Natl Acad Sci USA. 2010;107:3966-3971.
- Chitosan nanoparticles inhibit the growth of human hepatocellular carcinoma xenografts through an antiangiogenic mechanism. Anticancer Res. 2009;29:5103-5109.
- Progress and problems with the use of viral vectors for gene therapy. Nat Rev Genet. 2003;4:346-358.
- Gene therapy for Leber's congenital amaurosis is safe and effective through 1.5 years after vector administration. Mol Ther. 2010;18:643-650.
- Assessment of adenoviral vector safety and toxicity: report of the National Institutes of Health Recombinant DNA Advisory Committee. Hum Gene Ther. 2002, 13: 3-13. 10.1089/10430340152712629.
- A serious adverse event after successful gene therapy for X-linked severe combined immunodeficiency. N Engl J Med. 2003;348:255-256.
- Fatal systemic inflammatory response syndrome in a ornithine transcarbamylase deficient patient following adenoviral gene transfer. Mol Genet Metab. 2003;80:148-158.
- Human RPE65 gene therapy for Leber congenital amaurosis: persistence of early visual improvements and safety at 1 year. Hum Gene Ther. 2009;20:999-1004.
- Age-dependent effects of RPE65 gene therapy for Leber's congenital amaurosis: a phase 1 dose-escalation trial. Lancet. 2009;374:1597-1605.
- Subretinal delivery of recombinant AAV serotype 8 vector in dogs results in gene transfer to neurons in the brain. Mol Ther. 2008;16:916-923.
- Biodistribution of rAAV vectors following intraocular administration: evidence for the presence and persistence of vector DNA in the optic nerve and in the brain. Mol Ther. 2005;11:275-283.
- Periocular gene transfer of pigment epithelium-derived factor inhibits choroidal neovascularization in a human-sized eye. Hum Gene Ther. 2005;16:473-478.
- Adenoviral vector-delivered pigment epithelium-derived factor for neovascular age-related macular degeneration: results of a phase I clinical trial. Hum Gene Ther. 2006;17:167-176.
- Plasmid size up to 20 kbp does not limit effective in vivo lung gene transfer using compacted DNA nanoparticles. Gene Ther. 2006;13:1048-1051.
- Efficient non-viral ocular gene transfer with compacted DNA nanoparticles. PLoS One. 2006;1:e38-.
- Nanoparticles of compacted DNA transfect postmitotic cells. J Biol Chem. 2003;278:32578-32586.
- Nanoparticle-mediated expression of an angiogenic inhibitor ameliorates ischemia-induced retinal neovascularization and diabetes-induced retinal vascular leakage. Diabetes. 2009;58:1902-1913.
- A non-covalent peptide-based strategy for protein and peptide nucleic acid transduction. Biochim Biophys Acta. 2006;1758:384-393.
- Cell-penetrating peptide for enhanced delivery of nucleic acids and drugs to ocular tissues including retina and cornea. Mol Ther. 2008;16:107-114.
- Parker Read S, Cashman SM, Kumar-Singh R: A poly(ethylene) glycolylated peptide for ocular delivery compacts DNA into nanoparticles for gene delivery to post-mitotic tissues in vivo. J Gene Med. 12: 86-96. 10.1002/jgm.1415.
- Intracellular delivery of nanoparticles via the HIV-1 tat peptide. Nanomedicine (Lond). 2008;3:357-365.
- Nuclear localization of HIV-1 tat functionalized gold nanoparticles. IEEE Trans Nanobioscience. 2007;6:262-269.
- Tat peptide as an efficient molecule to translocate gold nanoparticles into the cell nucleus. Bioconjug Chem. 2005;16:1176-1180.
- The peptide carrier Pep-1 forms biologically efficient nanoparticle complexes. Biochem Biophys Res Commun. 2007;355:877-882.
- Nanoparticles for retinal gene therapy. Prog Retin Eye Res. 2010;29:376-97.
- Transfection of airway epithelium by stable PEGylated poly-L-lysine DNA nanoparticles in vivo. Mol Ther. 2003;8:936-947.
- Cell surface nucleolin serves as receptor for DNA nanoparticles composed of pegylated polylysine and DNA. Mol Ther. 2008;16:333-342.
- Gene delivery to mitotic and postmitotic photoreceptors via compacted DNA nanoparticles results in improved phenotype in a mouse model of retinitis pigmentosa. Faseb J. 2010;24:1178-1191.
- A partial structural and functional rescue of a retinitis pigmentosa model with compacted DNA nanoparticles. PLoS One. 2009;4:e5290-.
- Compacted DNA nanoparticles administered to the nasal mucosa of cystic fibrosis subjects are safe and demonstrate partial to complete cystic fibrosis transmembrane regulator reconstitution. Hum Gene Ther. 2004;15:1255-1269.
- Multi-institutional study of the angiogenesis inhibitor TNP-470 in metastatic renal carcinoma. J Clin Oncol. 1999;17:2541-2545.
- A Phase I and pharmacokinetic study of TNP-470 administered weekly to patients with advanced cancer. Clin Cancer Res. 1999;5:1989-1995.
- Safety and pharmacokinetic effects of TNP-470, an angiogenesis inhibitor, combined with paclitaxel in patients with solid tumors: evidence for activity in non-small-cell lung cancer. J Clin Oncol. 2002;20:4440-4447.
- Phase I trial of the angiogenesis inhibitor TNP-470 for progressive androgen-independent prostate cancer. Clin Cancer Res. 2001;7:1198-1203.
- Phase I dose escalation pharmacokinetics of O-(chloroacetylcarbamoyl) fumagillol (TNP-470) and its metabolites in AIDS patients with Kaposi's sarcoma. Cancer Chemother Pharmacol. 2000;46:173-179.
- Clinical and pharmacokinetic study of TNP-470, an angiogenesis inhibitor, in combination with paclitaxel and carboplatin in patients with solid tumors. Cancer Chemother Pharmacol. 2004;54:308-314.
- Targeting angiogenesis with a conjugate of HPMA copolymer and TNP-470. Nat Med. 2004;10:255-261.
- An orally delivered small-molecule formulation with antiangiogenic and anticancer activity. Nat Biotechnol. 2008;26:799-807.
- Broad Spectrum Anitangiogenic Therapy for Ocular Neovascularization [abstract]. IOVS. 2010.
- A PEDF N-terminal peptide protects the retina from ischemic injury when delivered in PLGA nanospheres. Exp Eye Res. 2006;83:824-833.
- Integrins: the keys to unlocking angiogenesis. Arterioscler Thromb Vasc Biol. 2008;28:1703-1713.
- In vitro and in vivo characterization of 64Cu-labeled Abegrin, a humanized monoclonal antibody against integrin alpha v beta 3. Cancer Res. 2006;66:9673-9681.
- Tumor-selective response to antibody-mediated targeting of alphavbeta3 integrin in ovarian cancer. Neoplasia. 2008;10:1259-1267.
- Randomized phase II study of cilengitide, an integrin-targeting arginine-glycine-aspartic acid peptide, in recurrent glioblastoma multiforme. J Clin Oncol. 2008;26:5610-5617.
- Targeting of ICAM-1-directed immunoliposomes specifically to activated endothelial cells with low cellular uptake: use of an optimized procedure for the coupling of low concentrations of antibody to liposomes. J Liposome Res. 2010.
- PLGA nanoparticle--peptide conjugate effectively targets intercellular cell-adhesion molecule-1. Bioconjug Chem. 2008;19:145-152.
- Identification of a potent peptide antagonist to an active laminin-1 sequence that blocks angiogenesis and tumor growth. Cancer Res. 2003;63:5060-5064.
- Nanoparticle-integrin antagonist C16Y peptide treatment of choroidal neovascularization in rats. J Control Release. 2010;142:286-293.
- Nanotechnology - Guided Imaging and Therapy of Retinal Vascular Disease [abstract]. IOVS. 2010.
- Targeted delivery of gemcitabine to pancreatic adenocarcinoma using cetuximab as a targeting agent. Cancer Res. 2008;68:1970-1978.
- Patra CR, Bhattacharya R, Mukhopadhyay D, Mukherjee P: Fabrication of gold nanoparticles for targeted therapy in pancreatic cancer. Adv Drug Deliv Rev. 62: 346-361. 10.1016/j.addr.2009.11.007.
- Cherukuri P, Glazer ES, Curley SA: Targeted hyperthermia using metal nanoparticles. Adv Drug Deliv Rev. 62: 339-345. 10.1016/j.addr.2009.11.006.
- Flt-1 intraceptors inhibit hypoxia-induced VEGF expression in vitro and corneal neovascularization in vivo. Invest Ophthalmol Vis Sci. 2005;46:1647-1652.
- The iron carrier transferrin is upregulated in retinas from patients with age-related macular degeneration. Invest Ophthalmol Vis Sci. 2006;47:2135-2140.
- Cell penetrating peptide POD mediates delivery of recombinant proteins to retina, cornea and skin. Vision Res. 2010;50:686-697.
- POD DNA Nanoparticles Rescue an Adult Mouse Model of Retinal Degeneration [abstract]. IOVS. 2010.