Application of microtechnologies for the vascularization of engineered tissues
Vascular Cell. 2011;
Received: 5 July 2011 | Accepted: 31 October 2011 | Published: 31 October 2011
Vascular Cell ISSN: 2045-824X
Abstract
Recent advances in medicine and healthcare allow people to live longer, increasing the need for the number of organ transplants. However, the number of organ donors has not been able to meet the demand, resulting in an organ shortage. The field of tissue engineering has emerged to produce organs to overcome this limitation. While tissue engineering of connective tissues such as skin and blood vessels have currently reached clinical studies, more complex organs are still far away from commercial availability due to pending challenges with
Keywords
microfabrication biomimetic approaches modular assemblyIntroduction
Progress in the development of large tissue-engineered organs has so far been limited by the inability to generate sophisticated three dimensional (3D) structures comprised of a functional vasculature. The vascular system is a dynamic environment comprised of a variety of cell types that constantly remodels itself under the influence of endothelial, immune, nervous and endocrine cells [1]. Vascular growth and remodeling are coupled with developmental and wound healing processes as well as the progression of various pathologies such as inflammation, cardiovascular diseases and cancer. Most of these processes depend on endothelial cells, which line the interior of blood vessels and form the endothelium. This interface between circulating blood and the surrounding tissues is responsible for proper solute transport and molecular exchange. It ensures the delivery of sufficient oxygen and nutrients to cells to maintain tissue homeostasis. Cells
The fabrication of tissue constructs often requires cell seeding of 3D scaffolds. These scaffolds are generally made of gels, foams or fibrous meshes and usually have basic macroscale properties that enable cell adhesion, migration and proliferation [4]. Although these properties are often sufficient to allow the formation of functional connective tissues such as skin [5, 6], bladder [7] and cornea [8, 9] and 3D tubular structures like blood vessels [10, 11] and urinary tract [12], most tissue engineering approaches still lack the capability to sustain the growth of thick engineered organs [13]. Skin and cartilage have been among the first engineered tissues ready for clinical applications since they do not require extensive internal vasculature to survive
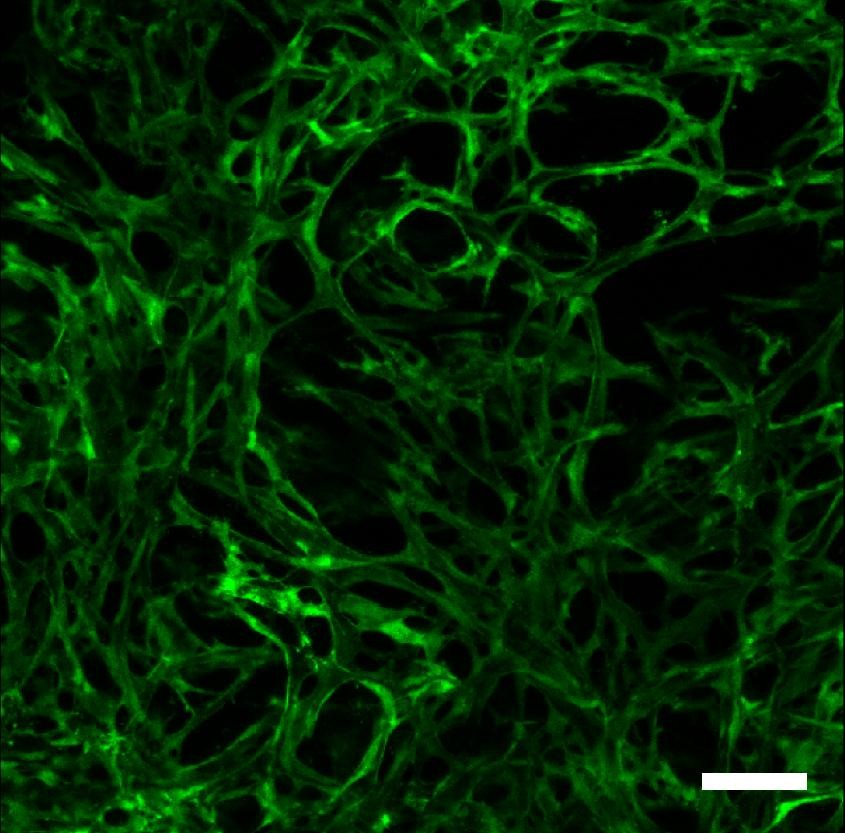
Figure 1
Figure 1 caption
Co-culture of fibroblasts and endothelial cells for one month in a collagen-GAG porous construct, resulting in a 3D capillary system within the biomaterial. Confocal imaging of the full thickness of the scaffold showing green fluorescent protein (GFP)-labeled endothelial cells forming vascular channels throughout the 3D structure of the biomaterial.
Microvascularization of Engineered Tissues through Angiogenesis and Inosculation
The need for adequate solute transport in cell-seeded scaffolds is essential for tissue survival and function. A key approach in attempting to induce the growth of a vascular network within 3D engineered tissue has been the incorporation of growth factors into the scaffolding material. It was shown that a macroporous scaffold, obtained by particle leaching, freeze drying or other pore forming technologies and functionalized with growth factors such as vascular endothelial growth factor (VEGF), basic fibroblast growth factor (bFGF) and platelet-derived growth factor (PDGF), can trigger the formation of vascular structures following
Both natural and synthetic scaffolding materials have been loaded with these pro-angiogenic molecules, leading to the sprouting of capillary beds within the constructs. However, the lack of directed growth of blood vessels to enable interconnectivity between the capillary networks still remains the biggest challenge of this technology.
Microvascular structures incorporated in tissue engineering scaffolds prior to their implantation can also be obtained by the co-culture of endothelial cells with the cell types of interest regarding tissue function. This cell-based approach uses the ability of endothelial cells to release growth factors and promotes the formation of capillaries
Similarly, Guillemette
The use of Microtechnologies to Engineer Vascularization in vitro
The application of microtechnologies to biomaterials can be used to reproduce the capillary network and allow the flow of culture medium through a construct during
Soft lithographic and micromolding processes have been used to create microfluidic devices consisting of branched networks that can be connected to perfusion systems
These properties are of utmost importance since they greatly influence the stability, oxygen and nutrient distribution and therefore the functionality of engineered tissues. Microfluidic systems can effectively transport solutes in capillary channels ranging from a few millimeters down to micrometers [38]. The control of fluid mechanics and mass transport over this wide range of dimensions has been used to study bioactive molecules and therapeutics in cardiovascular research and tissue engineering [39]. Sophisticated devices have recently been fabricated to reproduce a lung assist apparatus allowing the blood being perfused within the microchannels to be oxygenated by flowing through many parallel capillary-like channels analogous to the native lung architecture [40]. Although optimization of the gas transfer membrane and characterization of the blood flow in the device are still needed, this is a good example demonstrating the potential of microfabrication technologies to generate vascularized platforms for tissue engineering. Similarly, it was shown that microvascular cells could be seeded in the device to form a confluent endothelium on the walls of the vascular channels [41]. These studies demonstrated that microfabricated devices comprised of a fluidic network modeled on human vasculature can be successfully inosculated
Even though previous work has shown that microscale channels can be engineered
Biomimetic Approaches for Engineering Tissue Vascularization
Cells in the body are in contact with a complex 3D environment comprised of a combination of soluble factors as well as the ECM and basement membrane proteins found in the tissue in which they reside. Most tissues consist of multiple cell types organized into hierarchical structures that allow them to regulate their function. Modular tissue engineering, or bottom-up approaches, have recently emerged as powerful fabrication methods to generate 3D structures that mimic this organization and that recapitulate tissue structure and spatial resolution [51–54]. These techniques aim to generate biomimetic mesoscale structures by engineering microscale components and by using them as building blocks to fabricate larger tissue structures [55]. This approach has been used to control the cell microenvironment and the macroscale properties of relatively large and complex engineered tissues [56, 57].
Microgels are microscale hydrogels fabricated by merging microscale fabrication and hydrogel chemistry. They exhibit properties similar to native ECM, can sustain cell encapsulation and have tunable geometrical, mechanical and biological properties which make them excellent candidates for tissue engineering applications. Based on these characteristics, cell-laden microgels were fabricated and then assembled into 3D tissue constructs to create precise
microarchitecture containing repeated functional units that mimic the
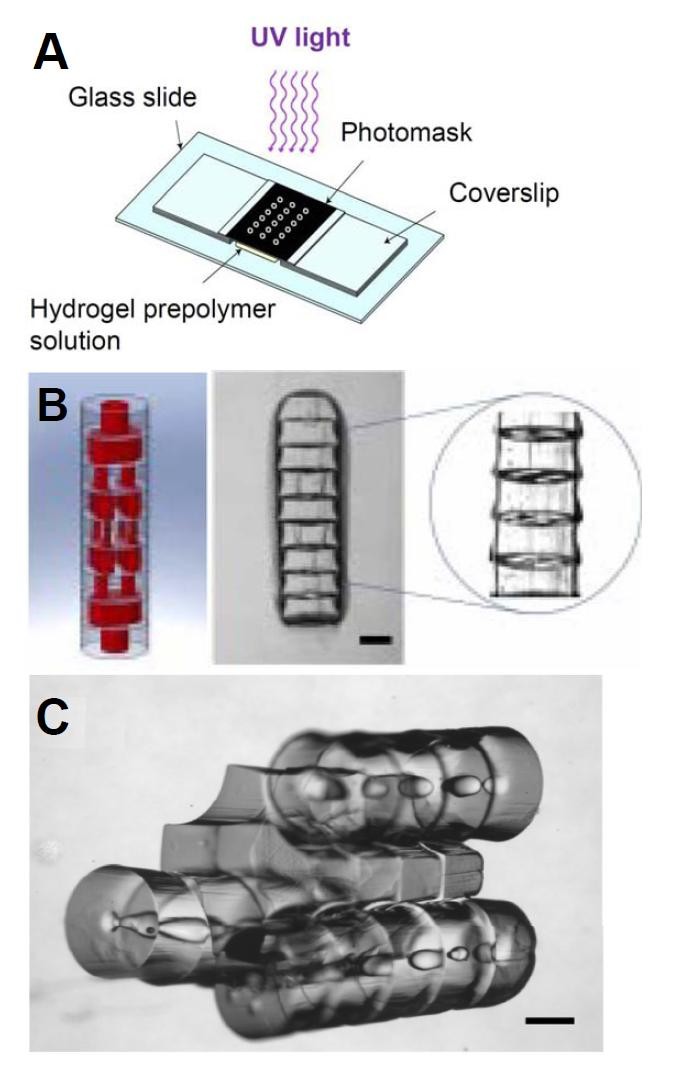
Figure 2
Figure 2 caption
Directed assembly of microgels using a modular approach. Schematic representation of a high-throughput photolithographic method (A). Design image of microgel arrays assembled into tubular structures embedded with 3D branching lumens and actual phase image of the microgel assembly after secondary crosslinking (B). Phase image of microgels following a sequential and directed assembly process (C). Scale bar: 500 μm. (Adaptation from Du ., Sequential assembly of cell-laden hydrogel constructs to engineer vascular-like microchannels, Biotechnol Bioeng, 2011, Copyright Wiley-VCH Verlag GmBH&Co. KGaA. Reproduced with permission.)
One of the challenges of directed self-assembly technology resides in the difficulty to scale-up the tissue produced. Larger structures have recently been built using lock-and-key principles and modular assembly [56]. Ongoing work is currently focusing on long-term perfusion aiming at developing mature and functional vasculature
Conclusions
One of the major limiting factors in the field of tissue engineering is the difficulty to generate functional 3D tissues due to the inability to integrate vascular structures into scaffolds. Building networks of vessels branched together into a complex interconnected structure connecting across multiple length scales remain one of the greatest challenges in tissue engineering. Most cells in the human body are within a few hundred microns from a capillary, allowing the delivery of adequate nutrients and supplies to the tissues and organs. Since most tissue engineering scaffolds are unable to provide such proximity for continuous solutes and oxygen flow, the engineering of large tissues severely lacks from diffusion and transport properties. The methods currently investigated to generate vasculature in scaffolds mainly involve the use of proangiogenic growth factors and cell-based approaches, which have shown promising results
Acknowledgements
This work was supported by the National Institutes of Health (EB008392; HL092836; HL099073; EB009196; DE019024), National Science Foundation (DMR0847287), the Institute for Soldier Nanotechnology, the Office of Naval Research, and the US Army Corps of Engineers. RG holds a postdoctoral fellowship from FQRNT and a scientific fellowship from DRDC-NSERC.
Authors’ original submitted files for images
Below are the links to the authors’ original submitted files for images.
Authors’ original file for figure 1
Authors’ original file for figure 2
References
- Engineering complex tissues. Tissue Eng. 2006;12:3307-3339.
- Engineering vascularized tissue. Nat Biotechnol. 2005;23:821-823.
- Tissue engineered vascular adventitia with vasa vasorum improves graft integration and vascularization through inosculation. Tissue Eng Part A. 2010;16:2617-2626.
- Advanced material strategies for tissue engineering scaffolds. Adv Mater. 2009;21:3410-3418.
- Wound tissue can utilize a polymeric template to synthesize a functional extension of skin. Science. 1982;215:174-176.
- Living tissue formed in vitro and accepted as skin-equivalent tissue of full thickness. Science. 1981;211:1052-1054.
- Tissue-engineered autologous bladders for patients needing cystoplasty. Lancet. 2006;367:1241-1246.
- Impact of cell source on human cornea reconstructed by tissue engineering. Invest Ophthalmol Vis Sci. 2009;50:2645-2652.
- Development of a reconstructed cornea from collagen-chondroitin sulfate foams and human cell cultures. Invest Ophthalmol Vis Sci. 2008;49:5325-5331.
- A completely biological tissue engineered human blood vessel. FASEB Journal. 1998;12:47-56.
- Tissue-engineered blood vessel for adult arterial revascularization. N Engl J Med. 2007;357:1451-1453.
- Tissue Engineering of a Genitourinary Tubular Tissue Graft Resistant to Suturing and High Internal Pressures. Tissue Eng. 2008;15:197-202.
- Tissue engineering--current challenges and expanding opportunities. Science. 2002;295:1009-1014.
- Cell-demanded liberation of VEGF121 from fibrin implants induces local and controlled blood vessel growth. Circ Res. 2004;94:1124-1132.
- Engineering vascular networks in porous polymer matrices. J Biomed Mater Res. 2002;60:668-678.
- In vitro reconstruction of a human capillary-like network in a tissue-engineered skin equivalent. Faseb J. 1998;12:1331-1340.
- Microfabrication Technology for Vascularized Tissue Engineering. Biomedical Microdevices. 2002;4:167-175.
- Polymeric system for dual growth factor delivery. Nat Biotechnol. 2001;19:1029-1034.
- Cell-demanded release of VEGF from synthetic, biointeractive cell ingrowth matrices for vascularized tissue growth. Faseb J. 2003;17:2260-2262.
- Biopolymeric delivery matrices for angiogenic growth factors. Cardiovasc Pathol. 2003;12:295-310.
- Enhancing the vascularization of three-dimensional porous alginate scaffolds by incorporating controlled release basic fibroblast growth factor microspheres. J Biomed Mater Res A. 2003;65:489-497.
- The effect of collagen-targeting platelet-derived growth factor on cellularization and vascularization of collagen scaffolds. Biomaterials. 2006;27:5708-5714.
- Normal Human Epithelial Cells Regulate the Size and Morphology of Tissue-Engineered Capillaries. Tissue Eng Part A. 2010;16:1457-68.
- A tissue-engineered endothelialized dermis to study the modulation of angiogenic and angiostatic molecules on capillary-like tube formation in vitro. Br J Dermatol. 2003;148:1094-1104.
- Inosculation of tissue engineered capillaries with the host's vasculature in a reconstructed skin transplanted on mice. Am J Transplant. 2005;5:1002-1010.
- Engineering vascularized skeletal muscle tissue. Nat Biotechnol. 2005;23:879-884.
- Transplantation of a tissue-engineered human vascularized cardiac muscle. Tissue Eng Part A. 2010;16:115-125.
- Engineered vascularized bone grafts. Proc Natl Acad Sci USA. 2010;107:3311-3316.
- Tissue-Engineered Vascular Adventitia with Vasa Vasorum Improves Graft Integration and Vascularization Through Inosculation. Tissue Eng Part A. 2010;16:2617-26.
- Tissue engineering: creation of long-lasting blood vessels. Nature. 2004;428:138-139.
- Influence of adult mesenchymal stem cells on in vitro vascular formation. Tissue Eng Part A. 2009;15:1751-1761.
- A macroporous hydrogel for the coculture of neural progenitor and endothelial cells to form functional vascular networks in vivo. Proc Natl Acad Sci USA. 2006;103:2512-2517.
- Human tissue-engineered blood vessels for adult arterial revascularization. Nat Med. 2006;12:361-365.
- Biodegradable microfluidics. Advanced Materials. 2004;16:2007-2012.
- Endothelialized microvasculature based on a biodegradable elastomer. Tissue Engineering. 2005;11:302-309.
- Fabrication of microfluidic hydrogels using molded gelatin as a sacrificial element. Lab Chip. 2007;7:720-725.
- Microfluidic scaffolds for tissue engineering. Nat Mater. 2007;6:908-915.
- The origins and the future of microfluidics. Nature. 2006;442:368-373.
- Microscale technologies for tissue engineering and biology. Proc Natl Acad Sci USA. 2006;103:2480-2487.
- Branched vascular network architecture: a new approach to lung assist device technology. J Thorac Cardiovasc Surg. 2010;140:990-995.
- Liver-assist device with a microfluidics-based vascular bed in an animal model. Ann Surg. 2010;252:351-357.
- Formation of perfused, functional microvascular tubes in vitro. Microvasc Res. 2006;71:185-196.
- Functional endothelialized microvascular networks with circular cross-sections in a tissue culture substrate. Biomed Microdevices. 2010;12:71-79.
- Three-Dimensional Microfluidic Tissue-Engineering Scaffolds Using a Flexible Biodegradable Polymer. Advanced Materials. 2006;18:165-169.
- Silk Fibroin Microfluidic Devices. Adv Mater. 2007;19:2847-2850.
- In vitro analysis of a hepatic device with intrinsic microvascular-based channels. Biomedical Microdevices. 2008;10:795-805.
- Towards organ printing: engineering an intra-organ branched vascular tree. Expert Opin Biol Ther. 2010;10:409-420.
- Rapid Fabrication of Bio-inspired 3D Microfluidic Vascular Networks. Advanced Materials. 2009;21:3567-3571.
- Laser assisted bioprinting of engineered tissue with high cell density and microscale organization. Biomaterials. 2010;31:7250-7256.
- A digital micro-mirror device-based system for the microfabrication of complex, spatially patterned tissue engineering scaffolds. J Biomed Mater Res A. 2006;77:396-405.
- Modular Tissue Engineering: Engineering Biological Tissues from the Bottom Up. Soft Matter. 2009;5:1312-1319.
- Fabrication of cell-containing gel modules to assemble modular tissue-engineered constructs. Nat Protoc. 2006;1:2963-2969.
- Design and fabrication of sub-mm-sized modules containing encapsulated cells for modular tissue engineering. Tissue Eng. 2007;13:1069-1078.
- Directed assembly of cell-laden microgels for fabrication of 3D tissue constructs. Proc Natl Acad Sci USA. 2008;105:9522-9527.
- Microengineered hydrogels for tissue engineering. Biomaterials. 2007;28:5087-5092.
- Micro-masonry: construction of 3D structures by microscale self-assembly. Adv Mater. 2010;22:2538-2541.
- Surfacedirected assembly of cell-laden microgels. Biotechnol Bioeng. 2010;105:655-662.
- Micromolding of shape-controlled, harvestable cell-laden hydrogels. Biomaterials. 2006;27:5391-5398.
- Guided and fluidic self-assembly of microstructures using railed microfluidic channels. Nat Mater. 2008;7:581-587.
- Vascularized organoid engineered by modular assembly enables blood perfusion. Proc Natl Acad Sci USA. 2006;103:11461-11466.
- Sequential assembly of cell-laden hydrogel constructs to engineer vascular-like microchannels. Biotechnol Bioeng. 2011;108:1693-703.
- Patterned differentiation of individual embryoid bodies in spatially organized 3D hybrid microgels. Adv Mater. 2010;22:5276-5281.