Growth factor purification and delivery systems (PADS) for therapeutic angiogenesis
Vascular Cell. 2015;
Received: 25 August 2014 | Accepted: 16 December 2014 | Published: 24 January 2015
Vascular Cell ISSN: 2045-824X
Abstract
Background
Therapeutic angiogenesis with vascular endothelial growth factor (VEGF), delivered either via recombinant protein infusion or via gene therapy, has shown promise in preclinical models of various diseases including myocardial infarction, renovascular disease, preeclampsia, and neurodegenerative disorders. However, dosing, duration of expression, and tissue specificity are challenges to VEGF gene therapy, and recombinant VEGF delivery suffers from extremely rapid plasma clearance, necessitating continuous infusion and/or direct injection at the site of interest.
Methods
Here we describe a novel growth factor purification and delivery system (PADS) generated by fusion of VEGF121 to a protein polymer based on Elastin-like Polypeptide (ELP). ELP is a thermally responsive biopolymer derived from a five amino acid repeat sequence found in human tropoelastin. VEGFPADS were constructed by fusion of the ELP coding sequence in-frame with the VEGF121 coding sequence connected by a flexible di-glycine linker.
Results
ELP fusion allowed for recombinant expression and simple, non-chromatographic purification of the ELP-VEGF121 chimera in yields as high as 90 mg/L of culture and at very high purity. ELP fusion had no effect on the VEGF activity, as the VEGFPADS were equally potent as free VEGF121 in stimulating HUVEC proliferation, tube formation, and migration. Additionally, the VEGFPADS had a molecular weight five-fold larger than free VEGF121, which lead to slower plasma clearance and an altered biodistribution after systemic delivery
Conclusion
PADS represent a new method of both purification and
Keywords
Vascular endothelial growth factor Elastin-like polypeptide Drug delivery Therapeutic angiogenesis Purification and delivery systemBackground
Loss of VEGF signaling or increase in anti-angiogenic factors have been implicated in many diseases including preeclampsia [1], renovascular disease [2], and neurodegenerative diseases [3,4]. Therapeutic angiogenesis with supplemental VEGF administration has shown preclinical efficacy in multiple animal models [4-10]. However, due to its short plasma half-life and susceptibility to degradation, exogenous VEGF must be continuously administered, often directly at the desired site of action, to achieve therapeutic benefit. The goal of this study is to characterize a biopolymer fusion with human VEGF. Fusion with this biopolymer, a synthetic protein based on human elastin, allows for recombinant production of large amounts of the chimeric protein, very simple nonchromatographic purification, and reduced plasma clearance relative to free VEGF.
Supplemental VEGF has been supplied in several preclinical disease models by either direct administration of the recombinant protein or by gene therapy techniques. In a dog model of myocardial infarction, direct infusion of VEGF at the infarcted site improved blood flow and increased neovascular development [10]. However, daily infusion for 28 days directly at the site of the infarcted tissue was required. Similarly, continuous VEGF infusion into the myocardium for six weeks decreased the size of the ischemic zone and improved cardiac function in a swine model of myocardial infarction [8]. In renovascular disease, microvascular rarefaction associated with renal artery stenosis was associated with a marked reduction in bioavailable VEGF in the kidney [2], and intrarenal administration of VEGF improved renal function, increased microvessel density, and improved renal scarring in a swine model of renal artery stenosis [6]. In preeclampsia, increased production of the VEGF antagonist sFlt-1 has been shown to be a major driver of the maternal syndrome [11-14]. Direct administration of recombinant VEGF via continuous intraperitoneal infusion sequestered the increased circulating sFlt-1 and reduced the hypertension [5]. VEGF supplementation has also shown efficacy in preclinical models of neurodegenerative diseases. In spinocerebellar ataxia type I (SCA1), VEGF mRNA and protein levels were decreased in the Purkinje layer of SCA1 transgenic mice, and VEGF administration improved the cerebellar pathology and the motor function in these mice [4]. However, direct intracerebroventricular administration was required. Similarly, loss of hypoxia inducible VEGF in neural tissue in transgenic mice lead to degeneration of lower motor neurons, causing a syndrome similar to amyotrophic lateral sclerosis [3].
These results suggest value for supplemental VEGF therapy in a myriad of disease models. However, they also highlight several drawbacks to recombinant VEGF therapy. VEGF has a very short plasma half-life. In humans, a terminal half-life of 33.7 minutes was measured after a 20 minute infusion [15]. For this reason, continuous administration, often directly at the target site, is required for efficacy. To overcome this limitation, sustained release methods have been developed. VEGF-loaded microspheres were injected into hindlimb muscles in rats, and these constructs resulted in slow release of VEGF over a period of seven days and evidence of vascular remodeling at time points as long as 70 days after a single injection [16]. In another application, VEGF-loaded microspheres were incorporated into alginate hydrogels to generate an injectable, slow-release hybrid delivery system [17]. This strategy lead to sustained release of VEGF over 28 days and marked improvement in angiogenesis and limb-sparing in a mouse model of hindlimb ischemia. In a recent report, a degradable VEGF-releasing hydrogel was created by fusing VEGF to the coagulation factor fXIIIa which was then crosslinked into fibrin hydrogels [18]. This construct exhibited a controlled degradation and VEGF release over a four week period, and yielded improved angiogenesis, perfusion, and healing in hind limb ischemia and in wound healing models.
In addition to its rapid clearance, recombinant VEGF produced in bacteria or yeast must be purified by a labor-intensive chromatographic protocol [19,20]. In a yeast expression system, yields of 40 mg of VEGF121 per L of culture have been reported using a nickel chromatography purification protocol [20]. However, chromatographic purification protocols are challenging to scale up to therapeutic production capacity, and the tooling required to do so contributes to increasing the manufacturing cost of goods (COGs).
Here we have developed a VEGF purification and delivery system (VEGFPADS) achieved by fusion of VEGF to a thermally responsive biopolymer via a flexible diglycine linker. Elastin-like polypeptide (ELP) is a genetically engineered protein consisting of a five amino acid repeating sequence [21]. This polypeptide has several unique properties that make it useful as a therapeutic delivery platform. First, it is thermally responsive, existing as a soluble protein below a characteristic transition temperature but self-associating into aggregates above that transition temperature [22]. This aggregation process is fully reversible. Second, ELPs can be expressed in bacterial recombinant expression systems, and they are easily purified due to their thermally responsive properties [23,24]. After recombinant expression and cellular lysis, ELP can be specifically separated from the soluble bacterial lysate by simply heating the solution or increasing the salt concentration in order to trigger ELP aggregation. Repeated centrifugation steps at temperatures above the ELP transition temperature leads to isolation of very pure preparations of the polypeptide. Third, because they are genetically engineered, the ELP sequence is easily modified to achieve the desired ELP size and transition temperature [22], and therapeutic proteins or peptides and targeting agents are easily fused to the ELP gene to create chimeric therapeutics [24-29]. Finally, because of its large size and its biocompatibility, ELP has many properties desired in a drug delivery vector, including a long plasma half-life [26,30], low immunogenicity [31,32], and biodegradability.
Here we show, using a simple non-chromatographic purification protocol, isolation of VEGFPADS as highly purified protein in yields up to 90 mg/L of bacterial culture. The purification requires only a warm water bath and a centrifuge and can be completed in 4 – 6 hours. The VEGFPADS retained full VEGF activity as assessed using endothelial cell proliferation, migration, and tube formation assays. Also, VEGFPADS exhibited a slower renal clearance and an altered biodistribution relative to unconjugated VEGF. We believe VEGFPADS, or growth factor PADS in general, represent a new, simple way to purify recombinant growth factors. They have the potential, either as stand alone agents or in combination with the novel controlled release methods recently described, to function as stabilized agents for therapeutic angiogenesis.
Methods
Generation of constructs
The coding sequence for VEGF121 was synthesized with codons optimized for expression in
Purification of VEGFPADS
pET25b + vectors containing the VEGFPADS coding sequence were transformed into
Cell culture
Human umbilical vein endothelial cells (HUVECs) were obtained from ATCC and maintained in M200 medium plus low serum growth supplement (Life Technologies) in a humidified 37°C incubator at 5% CO2. All experiments were performed on cells with <10 passages in culture. Cells were removed from flasks by trypsinization and counted using a Scepter® hand held cytometer (Millipore).
HUVEC proliferation assay
HUVECs were plated in 96 well plates (10,000 cells/well). Cells were serum and growth factor starved for 24 h in M200 medium without supplements then exposed to the indicated concentration of VEGF121 (ProSpec) or VEGFPADS for 72 h. Cell number was determined using the MTS aqueous cell proliferation assay (Promega). Experiments were performed in quadruplicate, and the data represent the mean ± s.e. of 3 independent experiments.
HUVEC tube formation assay
Sterile, non-tissue culture treated 24 well plates were coated with growth factor reduced Matrigel (BD Biosciences). 50,000 growth factor starved HUVECs were added per well in M200 growth medium + 0.1 mg/mL heparin without serum growth supplements, and PBS vehicle control, ELP control, VEGF121, or VEGFPADS were applied at a final concentration of 20 nM. Cells were incubated for 6 h at 37°C, then cells were imaged with an inverted brightfield microscope and 10× magnification objective. Five non-overlapping fields were imaged per well, and the number of tubes per field were counted and averaged for each well. Only tubes connecting two cell nodes were counted. Data represent the mean ± s.e. of three independent experiments.
HUVEC migration assay
HUVECs (30,000 cells/well) were placed in the upper well of Boyden chambers with 8 μm membrane pores coated with Matrigel (Corning BioCoat™) in M200 medium + 1% fetal bovine serum + 0.1 mg/mL heparin. The lower chamber contained identical medium plus PBS vehicle control, ELP control, VEGF121, or VEGFPADS at a final concentration of 10 nM or 50 nM. Cells were incubated for 16 h at 37°C. The cells on the upper surface of membranes were scratched off using cotton Q-tips. Membranes were removed, stained with 0.1% crystal violet in 10% ethanol, and the number of cells on the lower membrane surface were counted in four independent fields per membrane. Experiments were performed in duplicate, and data represent the mean ± s.e. of three independent experiments.
Polypeptide labeling
VEGF121 (ProSpec) or VEGFPADS were dissolved at 100 μM in 0.1 M NaHCO3 buffer, pH 8.3, and Alexa Fluor 633® succininimidyl ester (Life Technologies) was added to a final concentration of 300 μM. The reaction was allowed to proceed for 1 h at room temperature, then unreacted dye was removed by multiple washes with an Amicon 3,000 molecular weight cutoff spin filter (Millipore). Labeling efficiency was determined spectrophotometrically using a method modified from [24]. Removal of unreacted label was confirmed by TCA precipitation of the labeled protein and assessing the free fluorophor levels in the supernatant spectrophotometrically.
Pharmacokinetics and biodistribution
All animal use was approved by the Institutional Animal Care and Use Committee at the University Of Mississippi Medical Center and was carried out in accordance with the National Institutes for Health Guide for the Care and Use of Laboratory Animals. All procedures were carried out under full surgical isoflurane anesthesia. C57Bl/6 mice were catheterized in the femoral artery, and 123 nmol/kg AlexaFluor 633 – labeled VEGF121 or VEGFPADS were injected in the opposite femoral vein. Blood was sampled intermittently for a period of four hours. Four hours after injection, the animals were euthanized and the tissues removed for
Plasma fluorescence was determined by direct measurement of the fluorescence intensity of 2 μL plasma samples with 610 nm excitation and 660 nm emission using a fluorescence plate reader and a Nanoquant® plate (Tecan). Standard curves of the injected proteins were produced using known quantities of the injectate, and standards were scanned using the same scan settings as were used for plasma samples. Plasma fluorescence intensity was fit to the standard curves to determine the molar plasma concentration at each time point, and data were averaged for all animals (n = 4 mice per group) and represented as mean ± s.d. Averaged plasma clearance data were fit to a two compartment pharmacokinetic model as described previously [25].
Whole organ
Statistical analysis
Proliferation data were assessed using a two way ANOVA for polypeptide agent and concentration factors, and a Bonferroni multiple comparison was performed. Tube formation and migration data were assessed using a one-way ANOVA with a
Results and discussion
Purification and activity of VEGFPADS
The coding sequence for VEGF121 was cloned into a pET expression vector in frame with the ELP coding sequence to enable recombinant production. The chimeric ELP-fused VEGF (VEGFPADS) was purified by taking advantage of the thermally responsive property of the ELP moiety. After bacterial lysis, the VEGFPADS were separated from other soluble proteins by increasing the salinity of the solution and raising the temperature, which induces a reversible aggregation of the ELP domain. Centrifugation under these conditions selectively precipitated the VEGFPADS, and they were re-solubilized by mixing in cold phosphate buffered saline. As shown in Figure 1a, three thermal precipitation cycles were sufficient to produce highly purified VEGFPADS, and they migrated on a polyacrylamide gel at approximately the expected molecular weight. This method routinely yielded at least 90 mg of VEGFPADS per liter of bacterial culture, and the entire purification protocol from lysis to pure protein could be accomplished in less than one day.
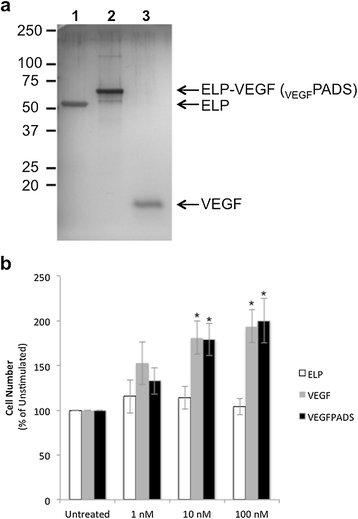
Figure 1
Figure 1 caption
Purification and activity ofPADS. a. SDS-PAGE gel with silver staining demonstrating the purity of PADS and ELP control polypeptides. Lane 1, ELP; Lane 2, PADS; Lane 3, VEGF. b. HUVEC cell proliferation was determined after 72h exposure to ELP, VEGF, or PADS at the indicated concentrations using the MTS cell proliferation assay. *Statistically significant increase versus untreated cells (p = 0.0003, two-way ANOVA with post-hoc Bonferroni multiple comparison).
We next assessed whether the VEGFPADS maintained their activity. It is possible that fusion of VEGF to the larger ELP domain could affect its receptor binding and reduce or eliminate its potency. To assess whether VEGFPADS could stimulate proliferation of endothelial cells, HUVECs in culture medium in the absence of growth factors were exposed to a concentration range of either free VEGF or VEGFPADS. VEGFPADS were very potent stimulators of HUVEC proliferation, achieving significant enhancement of proliferation rate at a concentration of as low as 10 nM (Figure 1b). Importantly, the potency of VEGFPADS was equivalent to that of free recombinant VEGF121 in this proliferation assay.
We also tested the ability of VEGFPADS to stimulate endothelial cell tube formation and migration. Tube formation was assessed using a growth factor reduced Matrigel assay. When plated on growth factor reduced Matrigel, HUVECs did not efficiently form tubes (Figure 2a, left panel). However, when the medium was supplemented with either free VEGF121 or VEGFPADS, tube formation was stimulated (Figure 2a, right panels). The ELP protein alone without VEGF fusion had no effect on tube formation. Tubes were counted for each treatment group, and this analysis revealed that VEGFPADS were equally as potent as free VEGF121 at stimulating HUVEC tube formation (Figure 2b). In addition to tube formation, VEGFPADS also stimulated HUVEC migration. As shown in Figure 3a, both free VEGF121 and VEGFPADS stimulated HUVEC migration through Matrigel in a Boyden chamber invasion assay. Both proteins stimulated migration at concentrations as low as 10 nM and produced equipotent and statistically significant migration at 100 nM (Figure 3b).
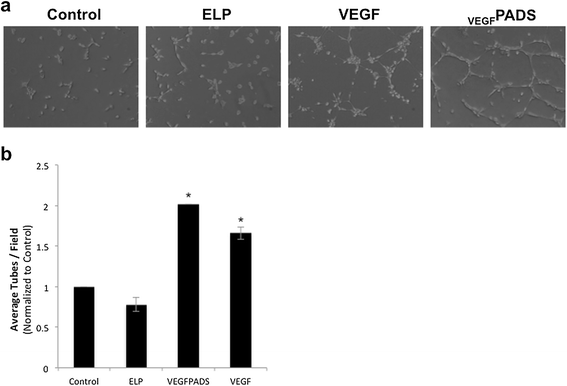
Figure 2
Figure 2 caption
PADS stimulate tube formation in HUVECs. a. HUVEC tube formation was assessed 6 h after seeding on growth factor reduced Matrigel and supplementing the media with 20 nM ELP, VEGF, or PADS. b. Average tubes per field were counted for six fields per sample. Data represent the mean ± se of four independent experiments. *p = 0.000003, one-way ANOVA with post-hoc Bonferroni multiple comparison.
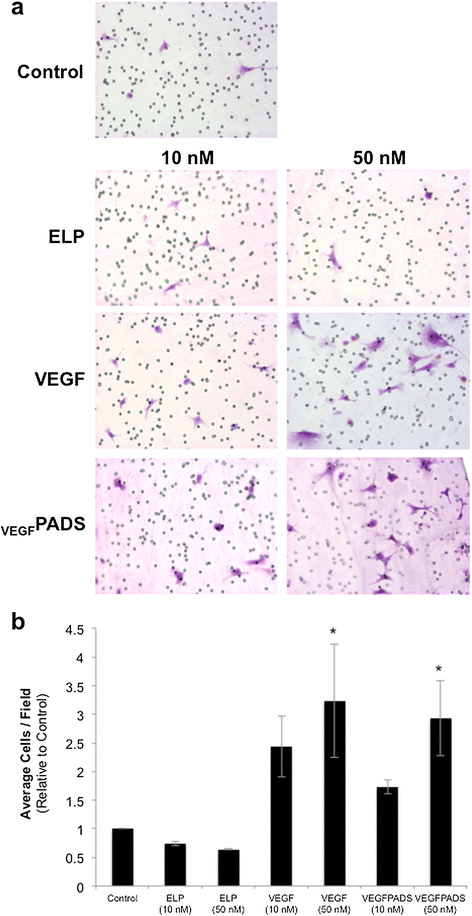
Figure 3
Figure 3 caption
PADS stimulate HUVEC migration. a. HUVEC migration was assessed 16 h after seeding in the top chamber of Matrigel-coated Boyden chambers in minimal media and supplementing the bottom chamber minimal media with ELP, VEGF, or PADS at the indicated concentrations. b. Average cells per field were counted for four to seven fields per sample. Data represent the mean ± se of three independent experiments. *p = 0.002, one-way ANOVA with post-hoc Bonferroni multiple comparison.
Pharmacokinetics and biodistribution of VEGFPADS versus free VEGF
In addition to examining the VEGFPADS activity
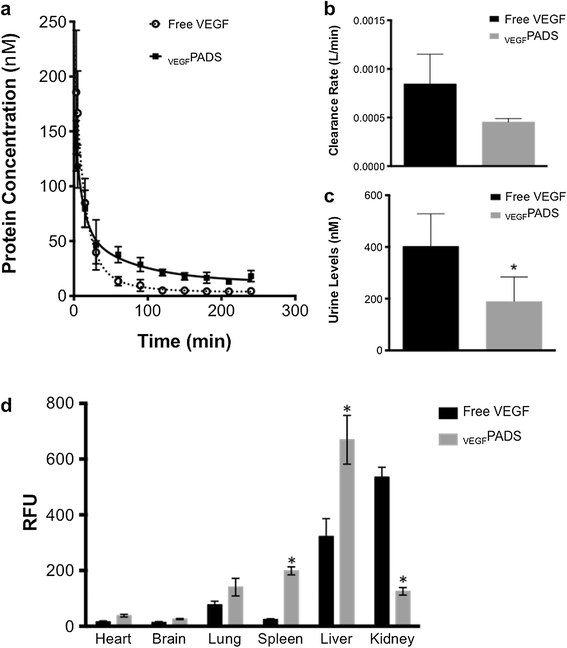
Figure 4
Figure 4 caption
PADS pharmacokinetics and biodistribution. a. Fluorescently labeled free VEGF or PADS were administered by IV injection to C57/Bl6 mice. Plasma levels were determined by direct fluorescence quantitation and fit to a two-compartment pharmacokinetic model. b. PADS had a slower plasma clearance rate than free VEGF, as was evidenced by lower levels in the urine at the end of the experiment c. Data represent the mean ± sd of four mice per group. *p = 0.03, Student’s t-test. d. ELP fusion significantly altered the biodistribution of VEGF, increasing its levels in the spleen and liver and reducing its levels in the kidney. *p <0.05, two-way ANOVA with post-hoc Bonferroni multiple comparison.
Table 1
Pharmacokinetic parameters of VEGF121andVEGFPADS
Free VEGF | VEGFPADS | |||
---|---|---|---|---|
Central compartment volume of distribution | (L) | 0.013 ± 0.005 | 0.013 ± 0.003 | |
Plasma clearance | (L min-1) | 0.0008 ± 0.0003 | 0.0004 ± 0.0004 | |
Area under curve | (nmol min L-1) | 3,863.8 ± 1,444.6 | 5,059.0 ± 528.5 | |
Distribution half life | (min) | 8.6 | 7.2 | |
Terminal half life | (min) | 30.2 | 52.4 |
We also confirmed that the pharmacokinetic and biodistribution results were not influenced by release of the dye from the carrier or by significant VEGFPADS degradation. Using SDS-PAGE analysis of VEGFPADS incubated in plasma at 37°C
In summary, by fusing VEGF to an engineered polypeptide carrier, we created a chimeric protein that is very easily purified from a recombinant expression system and maintains full VEGF activity as assessed in human endothelial cells. The VEGFPADS system allows for production of gram quantities of the recombinant growth factor at very high purity with a very simple purification scheme. This could represent a mechanism to facilitate the production of growth factors in a fast and inexpensive manner to make them more accessible for research purposes or to produce them at the scale needed for therapeutics. The system can easily be modified for the production of other growth factors, and we are in the process of generating other VEGF isoforms as ELP fusions as well as other GFPADS using several growth factors of interest for various diseases. In addition to maintaining their signaling ability, VEGFPADS also showed extended plasma life and altered biodistribution compared to the free growth factor. Given their ease of production, their potency, and their increased
We are currently evaluating the therapeutic efficacy of VEGFPADS in several disease models in which decreased VEGF levels have been implicated. For example, in preeclampsia, a major contributor to the maternal hypertension and other symptoms is the VEGF antagonist sFlt-1 [11]. We have recently described the ability of the ELP carrier to prevent placental transfer and fetal exposure of attached cargo [34], and we are evaluating the ability of VEGFPADS to bind the excess sFlt-1 and restore the available VEGF levels while preventing fetal exposure to VEGF in a rodent preeclampsia model. Also, VEGF has been demonstrated to increase microvascular density and partially restore renal function in a swine model of renovascular hypertension [6]. We are currently evaluating the ability of VEGFPADS to increase the VEGF bioavailability after systemic or direct intrarenal administration and restore or preserve renal function. Finally, VEGF has been shown to be reduced in the Purkinje layer of the cerebellum in the neurodegenerative disease SCA1 [4]. We are currently evaluating the brain deposition, clearance kinetics, and therapeutic efficacy of VEGFPADS versus unconjugated VEGF in a genetically engineered mouse model of SCA1.
Conclusion
The work presented here establishes VEGFPADS as easily produced, highly active modifications of recombinant growth factors. The ELP system is easily amenable to modification with any desired proteinacious therapeutic, and thus VEGFPADS are but one example of a therapeutic that can be generated using the ELP-based PADS. Given the ease of purification and the
Acknowledgements
Direct funding for this work was provided by National Institutes of Health (NIH) grant R01HL121527 to G.L.B. Partial salary support for E.G. was provided by NIH grant R00HL116774.
Additional file
Protein Stability and Dye Release in Plasma. a. The rate of degradation of VEGFPADS was determined after incubation for various times in mouse plasma at 37°C by SDS-PAGE analysis. b. The percentage of the total band intensity less than 50 kDa was determined for each time point (left axis). Also, dye release was detected by measuring the total plasma fluorescence before and after TCA precipitation of the protein component (right axis).
References
- Selective deficit of angiogenic growth factors characterises pregnancies complicated by pre-eclampsia. Br J Obstet Gynaecol. 1999;106:1019-22.
- Simvastatin promotes angiogenesis and prevents microvascular remodeling in chronic renal ischemia. FASEB J Off Publ Fed Am Soc Exp Biol. 2006;20:1706-8.
- Deletion of the hypoxia-response element in the vascular endothelial growth factor promoter causes motor neuron degeneration. Nat Genet. 2001;28:131-8.
- Vascular endothelial growth factor ameliorates the ataxic phenotype in a mouse model of spinocerebellar ataxia type 1. Nat Med. 2011;17:1445-7.
- Recombinant vascular endothelial growth factor 121 infusion lowers blood pressure and improves renal function in rats with placentalischemia-induced hypertension. Hypertension. 2010;55:380-5.
- Reversal of renal dysfunction by targeted administration of VEGF into the stenotic kidney: a novel potential therapeutic approach. Am J Physiol Ren Physiol. 2012;302:F1342-50.
- Therapeutic potential of VEGF and VEGF-derived peptide in peripheral neuropathies. Neuroscience. 2013;244:77-89.
- Magnetic resonance mapping demonstrates benefits of VEGF-induced myocardial angiogenesis. Nat Med. 1995;1:1085-9.
- Therapeutic angiogenesis. A single intraarterial bolus of vascular endothelial growth factor augments revascularization in a rabbit ischemic hind limb model. J Clin Invest. 1994;93:662-70.
- Angiogenic-induced enhancement of collateral blood flow to ischemic myocardium by vascular endothelial growth factor in dogs. Circulation. 1994;89:2183-9.
- Excess placental soluble fms-like tyrosine kinase 1 (sFlt1) may contribute to endothelial dysfunction, hypertension, and proteinuria in preeclampsia. J Clin Invest. 2003;111:649-58.
- Vascular endothelial growth factor ligands and receptors that regulate human cytotrophoblast survival are dysregulated in severe preeclampsia and hemolysis, elevated liver enzymes, and low platelets syndrome. Am J Pathol. 2002;160:1405-23.
- Elevated serum soluble vascular endothelial growth factor receptor 1 (sVEGFR-1) levels in women with preeclampsia. J Clin Endocrinol Metab. 2003;88:2348-51.
- Overexpression of the soluble vascular endothelial growth factor receptor in preeclamptic patients: pathophysiological consequences. J Clin Endocrinol Metab. 2003;88:5555-63.
- A target-mediated model to describe the pharmacokinetics and hemodynamic effects of recombinant human vascular endothelial growth factor in humans. Clin Pharmacol Ther. 2002;72:20-32.
- Daugherty AL, Rangell LK, Eckert R, Zavala-Solorio J, Peale F, Mrsny RJ. Sustained release formulations of rhVEGF165 produce a durable response in a murine model of peripheral angiogenesis. Eur J Pharm Biopharm Off J Arbeitsgemeinschaft Für Pharm Verfahrenstechnik EV. 2011;78:289–97. doi:10.1016/j.ejpb.2011.03.011.
- Sequential delivery of TAT-HSP27 and VEGF using microsphere/hydrogel hybrid systems for therapeutic angiogenesis. J Control Release Off J Control Release Soc. 2013;166:38-45.
- Long-lasting fibrin matrices ensure stable and functional angiogenesis by highly tunable, sustained delivery of recombinant VEGF164. Proc Natl Acad Sci U S A. 2014;111:6952-7.
- Prokaryotic expression, purification and activity assay of recombinant vascular endothelial growth factor. Nan Fang Yi Ke Da Xue Xue Bao. 2006;26:1263-8.
- A novel method to purify recombinant vascular endothelial growth factor (VEGF121) expressed in yeast. Biochem Biophys Res Commun. 1995;215:750-6.
- The synthetic polypentapeptide of elastin coacervates and forms filamentous aggregates. Biochim Biophys Acta. 1974;371:597-602.
- Temperature of Polypeptide Inverse Temperature Transition Depends on Mean Residue Hydrophobicity. J Am Chem Soc. 1991;113:4346-8.
- Purification of Recombinant Proteins by Fusion with Thermally Responsive Polypeptides. Nat Biotechnol. 1999;17:1112-5.
- Application of thermally responsive polypeptides directed against c-Myc transcriptional function for cancer therapy. Mol Cancer Ther. 2005;4:1076-85.
- A thermally targeted c-Myc inhibitory polypeptide inhibits breast tumor growth. Cancer Lett. 2012;319:136-43.
- Thermally targeted delivery of a c-Myc inhibitory polypeptide inhibits tumor progression and extends survival in a rat glioma model. PLoS One. 2013;8:e55104-.
- A thermally targeted peptide inhibitor of symmetrical dimethylation inhibits cancer-cell proliferation. Peptides. 2010;31:834-41.
- Inhibition of ovarian cancer cell proliferation by a cell cycle inhibitory peptide fused to a thermally responsive polypeptide carrier. Int J Cancer. 2010;126:533-44.
- Application of thermally responsive elastin-like polypeptide fused to a lactoferrin-derived peptide for treatment of pancreatic cancer. Molecules. 2009;14:1999-2015.
- Tumor accumulation, degradation and pharmacokinetics of elastin-like polypeptides in nude mice. J Control Release. 2006;116:170-8.
- Biocompatibility of elastin-like polymer poly(VPAVG) microparticles: in vitro and in vivo studies. J Biomed Mater Res A. 2006;78:343-51.
- Development and characterization of a fusion protein between thermally responsive elastin-like polypeptide and interleukin-1 receptor antagonist: sustained release of a local antiinflammatory therapeutic. Arthritis Rheum. 2007;56:3650-61.
- Hyperexpression of a synthetic protein-based polymer gene. Methods Mol Biol. 1997;63:359-71.
- George EM, Liu H, Robinson GG, Bidwell GL (2014) A polypeptide drug carrier for maternal delivery and prevention of fetal exposure. J Drug Target 1–13. doi: 10.3109/1061186X.2014.950666
- Tracking the in vivo fate of recombinant polypeptides by isotopic labeling. J Control Release. 2006;114:184-92.