A 3-Dimensional Hypothesis of Oxidative Phosphorylation
Vascular Cell. 2023;
Received: 15 November 2022 | Accepted: 22 December 2022 | Published: 27 March 2023
Vascular Cell ISSN: 2045-824X
Abstract
In general, hitherto, all scholarly illustrations of oxidative phosphorylation are schematically presented in two dimensions as in Fig.1, where the electron current is shown resulting in the transmembrane proton gradient driving the formation of ATP via the F1F0- ATPase [1]. In this hypothesis, the electrical gradient that results from electron transport induces a conformational change of the F1F0-ATPase coupled with a change of hydrogen bonding of a strategic shell of H₂O molecules, favouring the thermodynamics of the reaction ADP with inorganic phosphate (Pi) to produce ATP. This hypothesis presents the whole coupling process of the mitochondrial inner membrane in three dimensions, essential for this particulate biochemistry [1, 2].
Keywords
Mitochondrion Oxidative Phosphorylation Hydrogen-bonded water Honeycomb structuresIntroduction
Oxidative phosphorylation is the final stage of aerobic cellular respiration when molecular oxygen is reduced to H₂O [3]. It is composed of an electron transport chain coupled with the phosphorylation of ADP to produce ATP, the energy currency of the cell. Along the electron transport chain, the electrons pass from one macromolecular complex to the next and terminate at molecular oxygen. The reduction-oxidation (redox) potential generates a membrane potential across the inner mitochondrial membrane (IMM) which powers the formation of ATP by the F1F0 ATPase at three coupling sites along the chain I, III and IV. When the electrons are provided by succinate instead of NADH, coupling site 1 is by-passed resulting in a P/O ratio of approximately 2 [3]. In this day and age, any acceptable hypothesis of oxidative phosphorylation must be, above all, underpinned by the rules of thermodynamics: each segment of a bioenergetic reaction or prediction must be justifiable, feasible and entirely consistent with the laws of thermodynamics. Energy change is a characteristic feature of each and every chemical reaction and the sign and magnitude of free energy change (ΔG) of each consecutive step of the overall oxidative phosphorylation process must be identifiable before there can be clarity, an understanding and a complete analysis. What is clear is that the term energy transduction, if used, needs substantial clarification.
The unpredictable complexities of a macromolecular membrane topography, ultimately determined by protein quaternary structure, can only be left to the imagination at present. The research of Greer J and Bush BL (1978) has described a method that outlines steps to determine surface topography and although this work provides essential information relevant to the overall architecture and detailed macromolecular organisation of a cell membrane, the precise organisation of oxidative phosphorylation macromolecular structures embedded in the coupling membrane remains unresolved [4, 5]. High matrix density, staining and embedding have the disadvantage of obscuring internal detail and maybe loss of it [6]. Any freezing process must surely affect the solvation shells of proteins and probably their conformation, particularly if the macromolecules and water shell are to be considered the functional unit under discussion here. Even so, however impenetrable remain the complexities, with hitherto undiscovered techniques there should be an acceptable, detailed 3-D resolution of oxidative phosphorylation eventually; one that supports the hypothesis and does not conflict with any biological or chemical axiom. A structure-function model is proposed in this communication, and in the absence of precise biological evidence, an imaginative logical leap is taken to complete a comprehensive hypothesis that should be testable in due course. There will always be demand for the refinement of techniques that penetrate and elucidate the chemical reactions that constitute complex biochemistry, not least to investigate the remarkable properties of the solvent water that investigators in the field believe can be different inside the cell from bulk water [7, 8, 9]. Our knowledge of cellular water structure remains incomplete although there is a widespread belief that the dynamics of biological macromolecules in general, and proteins in particular, cannot be decoupled from that of their aqueous solvent. At present, there is no simple qualitative or quantitative account of how protein and solvent dynamics interact at a macro-molecular surface. Ball (2017), in his comprehensive review of the relationship between water and macromolecules, provides ample evidence that a vague sheath of hydration water exists in complex associations with macromolecules, and however daunting a task, the atomic resolution of that relationship now becomes essential [8]. The studies cited in Ball's review represent a selection from the vast amount of valuable research that supports the concept and the premise on which the hypothesis presented here is largely based.
The Role of H₂O-H₂O Hydrogen Bonding
Over a century ago, Rontgen (1892) postulated that liquid water was comprised of a high-density fluid and a low-density fluid while Bernal and Fowler proposed that the tetrahedral geometry of the water molecule could be responsible for this characteristic [10, 11]. Subsequently, it was Pople (1951) who proposed that water had distributions of hydrogen bonds and far-reaching evidence supporting this is presented by Brini et al. (2017) and Maestro et al. (2016) in reviews of the molecular structure and energies of water molecules [12, 13, 14]. Liquid water forms a fluctuating network of hydrogen bonds with an average lifespan of around one picosecond. One question asked is 'might the enthalpic and entropic consequences of water's ability to form relatively structured hydrogen-bond networks in small clusters and within cavities make it especially sensitive to the geometry or changing the geometry of macromolecular confinement for instance'? The hydrogen bond lifespan can vary significantly depending on location: a longer lifespan of hydrogen bonds occurs in deep clefts of a myoglobin molecule for example where macromolecular geometry changes have been identified [8]. Basically, if the hydrogen bonds between water molecules change from straighter and stronger to bent and weaker bonds, the water changes from one of low density to one of a higher density. Based upon the long-time belief that water exists as a mixture of high-density water (HDW) and low-density water (LDW), Pollack maintains that intracellular water can undergo abrupt phase changes that serve biological functions [15]. In the crowded cytoplasm, it is understood that the three-dimensional hydrogen-bonded network of H₂O molecules with five or six-membered rings plays a fundamental role. It is known that low-density water (LDW) has lower reactivity and is more ice-like whereas high-density water (HDW) is more disorganised and the more reactive of the two polymorphs. The strong possibility of the existence of LDW and HDW has encouraged many researchers, using a variety of techniques, to study the phenomenon and many properties investigated have been related to the interplay between the two interconverting structural polymorphs [16]. With evidence supporting the existence of water in two forms - the high-density form (HDW) and low-density form (LDW) - a variable ratio of LDW/HDW is very plausible such that spontaneous phase changes at any time could well be critical and a key factor that dictates solvation, molecular conformation and associations [9]. Phase changes will potentially affect biological and physical processes as the direction of any biochemical reaction is determined by or is dependent upon the free energy of hydration of each reactant involved. Furthermore, dynamic degrees of freedom in the hydration shell could provide fluctuations that assist proteins to undergo the conformational shifts intrinsic to their chemical function. One probable outcome of this is the vast variety of substrate-specific enzymes endowed with molecular structures hydrated by the most advantageous of two water structures, strategically selected and located at precise locations of macromolecules at a critical time, thereby mutually enhancing catalytic reactions [17, 18]. An enzyme-water mutualism is predicted by the author to participate in the ATP synthase of oxidative phosphorylation and this concept is implicated here. It is plausible that the evolutionary 'timing' of the proteins to bring their hydration environment closest to a beneficial phase transition presents a way to produce significant effects from small changes in a microenvironment; all delicately poised as perhaps for a voltage-sensitive membrane pore. A possible contribution to this in the minute confines of macromolecular environments could be the unique organising force of hydrophobicity, when hydrogen bonding is maximised between water molecules and the area of contact between non-polar solutes and water minimized [19, 20]. Macromolecular folding could result from this or, alternatively, a configuration change could induce a hydrophobic reaction and consequently a phase change of the water. Macromolecular folding relating to hydrophobic energies has been described by Chothia (1976) [21].
In the first place, this hypothesis rests heavily upon the understanding of the hydrogen bonding of water molecules and the enthalpic consequences brought about by changes in H2O-H₂O bonding due to the interplay of the two water polymorphs. Thermodynamics of solvation is generally governed by a balance between the enthalpic water-water and water-solute interactions providing the source of one of the key forces that dictate macromolecular conformations [8]. The latter envisages surfaces of proteins extending into the solvent and this coupling can make the hydration shell part of the molecule itself and play a role in intramolecular rearrangement and intermolecular recognition processes. So, protein treats water molecules as a resource to be exploited implying that the water molecules can mediate an interaction between a protein and substrate either to increase selectivity or enable recognition of multiple substrates. Some hydration water molecules may adopt well-defined positions around macromolecules with functional roles. Szent-Gyorgyi (1957) first proposed a biological function consisting of the building and destruction of water structures and that muscle contraction happens due to the collapse and reforming of hydrogen bonds of water molecules thereby directing alignment of the thick and thin muscle filaments: the prerequisite of contraction and relaxation [22]. He also proposed that water structures around dissolved macromolecules may have different crystalline structures dependent upon the polar or non-polar nature of the groups of that molecule [22]. By way of emphasis at this point, it is compelling to speculate on the evolutionary origin of sophisticated molecular and configurational changes due to intracellular water structure, and attention is drawn to amoeboid locomotion, a typical mode of cell migration [23]. Students of biology learn first that amoeboid movement is by means of a change of the cytoplasm from gel to sol. It is generally understood that plasma gel is converted to plasma sol causing the cytoplasmic slide. However, the mechanism underlying sol-gel transformation including any relevant trigger signal and regulating factors remains unclear and continues to be investigated. Evidently, from the report by Mitchison and Cramer (1996), a force is generated by macro-molecules coupled with LDW/HDW change [24]. What is known is that inside the amoeba there are proteins that can be activated to convert the gel into a more liquid state and Ca++ is said to be important for that gel-sol conversion. It appears actin filaments may have multiple roles in cell motility and this could well be governed in part by their hydration shell. The resulting increase in HDW (sol) with an elevation of H₂O activity provides pressure change [24].
The liquid polymorphs in rapidly exchanging equilibrium of the hydrogen-bonded H₂O clusters in two forms have relevance to this hypothesis. Each polymorph is endowed with different physical and solvent properties such that partitioning of a charged solute or group, in one or the other polymorph is dependent upon the size and electronic configuration. The polymorph properties determine the ease by which hydrogen bonds are broken preceding hydration by one water form or another. For example, in this regard, it is known that Na+ ions partition differently from K+ ions [9]. So, the solvent of choice determines the free energy of hydration of the species in question and the change of hydration energy if the polymorph transforms. The rapid interchange of HDW to LDW or vice versa results in free energy change (ΔG) dependent upon the alternative polymorph, which in turn is coupled with the hydration energy of any species solvating in either LDW or HDW. It has been shown that the free energies of hydrolysis of molecules like ATP are given by the difference between the free energies of hydration of products and reactants [25]. Based on this, when two contiguous polymorphs of water exist in the interstitium of a cell or matrix of mitochondria, a source of local free energy is provided coincident with a phase change and consequent change in the HDW/LDW ratio affecting the availability of H₂O molecules to solvate a specified solute or group and thus, in a sense, acts as a reservoir of energy. ΔGw is larger and more positive when LDW replaces HDW and reversed when HDW replaces LDW. This, in turn, accounts for the change in energy of hydration of any solute in either polymorph as detailed by Wiggins [26]. Furthermore, when such displacements incur and the thermodynamic cost is high enough, a protein molecule may change its configuration by folding if solvation and folding of a protein are considered a unitary process [26, 27, 28]. Predictably a folding or cycling of macromolecular events brought about by solute change or charged group preference for one or other polymorph has implications in cyclic enzyme action. This concept has also been developed and supported experimentally by Wiggins and considered here to have significance in relation to the reversible rotation of the γ-subunit of the F1F0-ATPase [9]. A decrease in light scatter of coupled mitochondria suspended in hypertonic KCl supports the view that depolarisation of the coupling membrane (by inward diffusion of K+ in this case), converts matrix LDW to HDW by a critical amount, uncouples and prevents ATP synthesis. In contrast to this, the potassium ionophore Valinomycin (Val) induces ATP synthesis in non-respiring mitochondria suspended in a K+-free medium [29]. The preferred interpretation here is that the ValK+ species unaccompanied by an internal anion diffuses from the matrix and this diffusion outward, limited by the build-up of external positive charge, results in hyperpolarisation of the coupling membrane. This increase in external positivity and hyperpolarisation is believed to cause a configurational change in the coupling membrane and consequent increase in the LDW/HDW ratio favouring ATP formation from ADP and inorganic phosphate. This is in contrast to the behaviour of mitochondria suspended in hypertonic KCl when depolarisation results in a decrease in the LDW/HDW ratio. In the past, mitochondria swelling studies have been extensive and linked to oxidative phosphorylation [30]. Subsequent studies have shown that what would have been interpreted in earlier studies as swelling could not be reconciled, in certain experiments, when changes in the actual size of mitochondria were determined using laser technology [31]. In these experiments, light scatter changes were recorded which could best be interpreted by invoking a change in the LDW/HDW ratio; the event considered here to be closely linked to oxidative phosphorylation.
As reviewed by Boyer in 1997, there are three pertinent catalytic sites on the F1F0-ATPase [32]. The first binds ADP and phosphate, then undergoes a conformational change providing for a tightly bound ATP and then a subsequent conformation change releases this ATP [32, 33]. These αβ changes come with a rotation of the inner core of the enzyme (γ subunit) driven by the protons crossing the mitochondrial membrane [34]. This rotation is a strong indicator that the oxidation phosphorylation process is part of a three-dimensional event involving protein conformational changes throughout the coupling membrane powered by a proton gradient or transmembrane proton motive force (Δp) resulting from electron transport. A coupled three-dimensional arrangement of the oxidation phosphorylation process is of fundamental importance to this hypothesis and not ostensibly two-dimensional as in Figure 1. The conformational changes identified ultimately release tightly bound ATP, supporting the widely held view now that the proton motive force is not powering ATP synthesis as directly as Mitchell predicted initially in 1961 [32, 35, 36, 37]. It is this author's belief that these are the conformational events which change LDW/HDW (low-density/high-density water ratio) providing the mysterious energy-requiring link between the rotation of α-subunit and ATP synthesis and release [37]. The increase in this ratio, when H₂O activity is reduced, will favour ATP hydration and synthesis at the F1-ATPase: thus, providing a feasible coupling mechanism which is more thermodynamically acceptable than a proton gradient somehow reversing the F1F0-ATPase directly. According to the literature, the transmembrane proton and electrochemical potential Δp from electron transport are mechanically transmitted to the hexamer of the αβ-subunits. The step-wise rotation of the F1/F0 complex is the result when ADP reacts with Pi to form ATP tightly bound in the first instance and a second configuration which allows ATP to be released [35, 38]. Apart from this, another crucial feature lacking a molecular description is how rotation driven by Δp is generated, and how rotation transmits energy into the catalytic sites of the enzyme to produce the stepping action during rotation [39]. In 1989, Tsuprun et al. suggested proton gradient transport, via the embedded membrane F0 sector, induces a conformational change in the stalk which in turn alters the catalytic centre of the F1-ATPase [40]. Whatever are the sequential conformational changes of the αβ -subunits and rotation of the γ subunits, the hypothesis submitted here provides a means whereby these are powered by LDW/HDW change, which may also act as a sink for the H+ gradient enabling it to dissipate across the coupling membrane. These reactions are thermodynamically sound; there is no infringement of any thermodynamic law and a true coupling of the oxidation reactions and phosphorylation takes place. In truly coupled chemical reactions the product of the 1st reaction must become a reactant of the 2nd. Here the product of the first, the electron transport reaction and proton gradient, results in the changed HDW/LDW ratio driving ATP formation from ADP and inorganic phosphate in the second reaction of the coupled sequence. According to Swanson et al. (2007), hydrogen-bonded chains of water molecules may comprise 'water wires' that support proton translocation through proteins and are perhaps controlled by protein motions [41]. Kaila et al. (2014) describe such a process in the initial step in the mitochondrial and bacterial respiratory sequence when a transient proton-conducting water channel is formed by complexes in the membrane [42]. The same authors conclude: water-gated transitions may provide a general mechanism for proton pumping in biological energy conversion enzymes [42]. Furthermore, Noji et al. (2017) reported an associated water molecule of a glutamic acid residue of the β subunit of the F1-ATPase that enhances proton transfer: all additional evidence in support of a sheath of water molecules functionally associated with protein subunits [43].
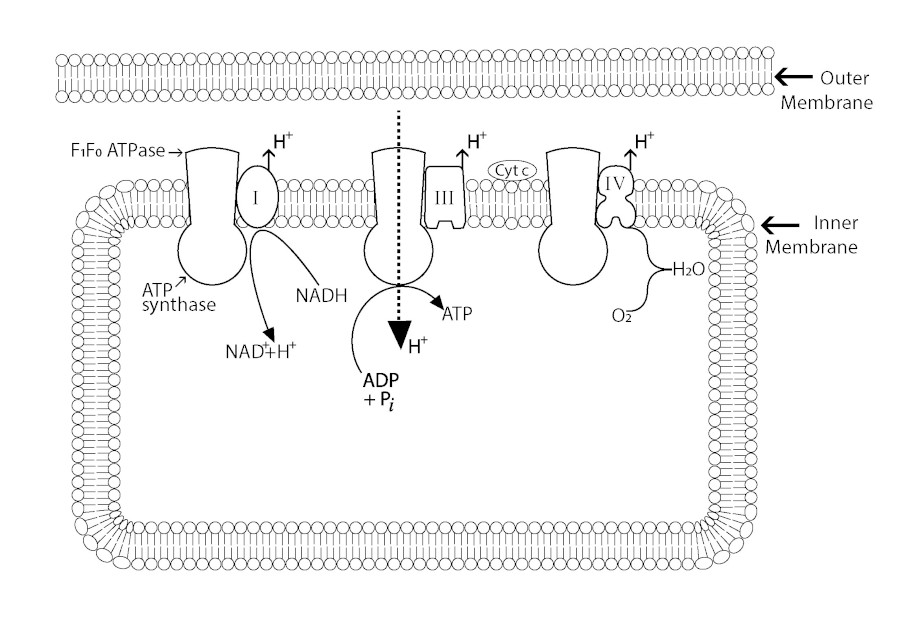
Figure 1
Figure 1
Mitochondrial oxidative phosphorylation in two dimensions.
In brief: A conformational change induced by charge separation is believed to alter the LDW/HDW ratio and when elevated, the reduced activity of H₂O favours ATP synthesis. The contention here is that ADP + Pi reacting to form ATP + H₂O is not thermodynamically feasible without a critical change in the LDW/HDW ratio that leads to a reduction in H₂O activity due to a configurational change of the F1F0-ATPase complex, or part of it. As a consequence of this the reversible ATPase/ATP synthase reaction:
ADP + Pi + 2H+ ⇌ ATP + H₂O + 2H
proceeds more favourably to the right with elevated LDW and is endothermic (+ ΔG). Conversely, elevated HDW favours the reaction to the left and is exothermic (- ΔG).
Accounting for three energy coupling sites along the electron transport chain
It is essential that the electron transport complexes, in setting up the transmembrane charge separation are juxtaposed with a macromolecular close-fit to the F1F0-ATP synthase. This close-fit is predicted to create the critical membrane potential change associated with three of the five ET complexes identified and the required conformational change of part of the ATP synthase that results in a shift of the HDW/LDW ratio of the solvation shell. A critical lower ratio will result in the formation of ATP tightly bound to a subunit of the F1F0-ATPase and a second conformation due also to the proton gradient releasing this ATP being favoured by a reversal of the HDW/LDW ratio change.
To whatever extent the structural components I, II, III, IV etc. have been characterised, conceivably, in their native state they fit neatly together pursuant to a gradual refinement in the evolutionary sense [30, 44, 45]. This organisational progression is envisaged to culminate in an efficient three-dimensional functioning unit of a membrane that becomes the tubular-like inner mitochondrial membrane (IMM). The chemiosmotic hypothesis, or any other, should be able to meet a 3-D requirement or it is invalidated as a functioning process delivering ATP from ADP and Pi via coupled reactions. Although the proton motive force is still favoured as an accepted component of the F1F0-ATP synthetase process, the initial membrane potential and H+ separation set-up must be reconcilable with a 3-dimensional functional macromolecular structure of the IMM [46]. The ATP-ase and redox respiratory chain must have a macro molecular architecture arranged in a repeating manner that fits functionally into the well-documented repeating membrane pattern identified regularly throughout the plane of the coupling membrane. An abundance of evidence from the earliest electron-microscopy images shows high and low regions of the coupling membrane such that there must be a contour in the plane of the membrane with peaks and troughs that could well be of biophysical significance. The repeating units of the inner mitochondrial membrane give the membrane regularity with undulations in the plane of the membrane. This means that there are thick and thin portions of the coupling membrane: the troughs providing regions of greater capacitance, more so than the peak regions of the membrane. Any critical density of charge will most certainly be located at the thin regions of the membrane. This would make the topography of the cristal membrane of mitochondria in some way analogous to that of the myelinated nerve and the benefit of thick and thin portions of a charge-separating cellular membrane may not be exclusive to those neurones. The critical potential created by electron transport is perceived by the author as a preponderance of the separated charge, if not all, held across the thinner regions of the coupling membrane and not those parts of the membrane occupied by the F1F0-ATPase. These parts of the membrane are too thick to act as the preferred effective condensers (indicated as a dashed circle in Figure 2) [46].
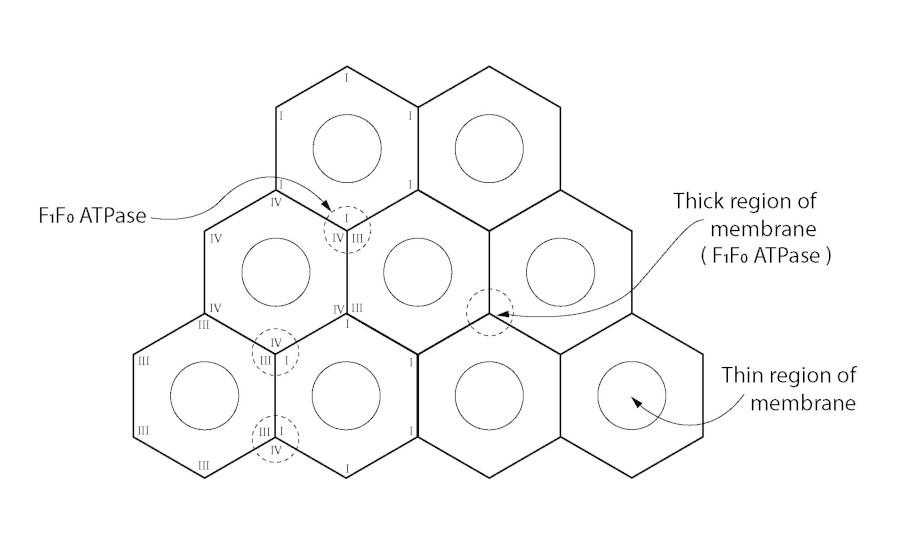
Figure 2
Figure 2
Sixfold symmetry structure of coupling membrane.
What protrudes from the membrane is an order of magnitude in nm that permit a close relationship or even binding of the electron transport complexes with the F1/F0-ATP synthetase. The frequently reported hexagonal lattice and threefold symmetry structures of cellular membranes, provide a structural option for a 3-dimensional 'fit' in the coupling membrane [5, 6, 47, 48, 49, 50, 51, 52]. Figure 2 is a diagram of the sixfold symmetry arrangement of coupling membrane components indicating thick and thin regions of the coupling membrane. Figure 3 shows how each threefold symmetry structure of F1F0-ATPase is located with coupling sites I, III and IV, juxtaposed. Amongst others, Letts and Sazanov (2017) provide evidence of a supercomplex where there is a close association between complex I and complex IV: further evidence of a three-dimensional arrangement [45].
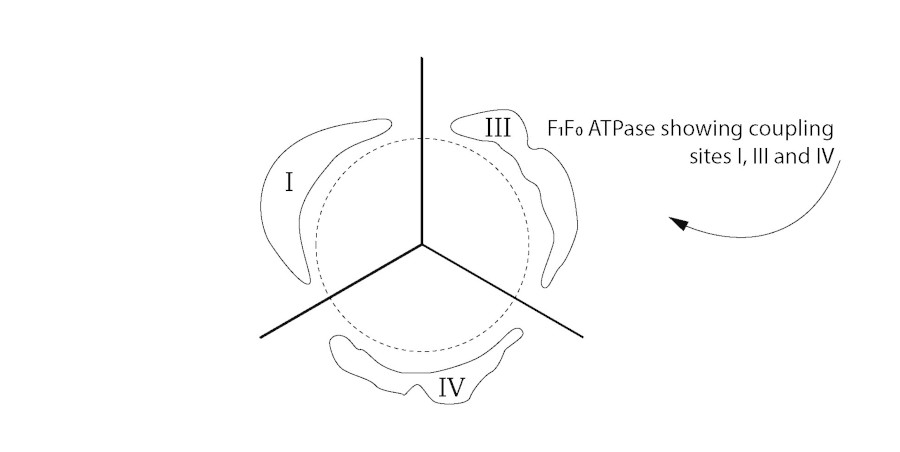
Figure 3
Figure 3
Threefold symmetry structures. Thick region of membrane.
The biogenesis and assembly of membrane complexes remain unexplained. How do the respiratory chain complexes and ATPase synthase assemble? There is evidence of double rows of ATP synthase in paramecium, for example, and these results suggest an evolution and ultimately an alignment to optimize efficiency in eukaryotes, more so than any fluid membrane could provide [53]. In addition, potato respirasomes differ from 3-dimensional model of bovine respirasomes, (Bultema et al. 2009) [54]. These authors remain undecided whether the 'string architecture' identified is conserved in eukaryotes or differs in different organisms. Even so, the precursor of the order in the coupling inner mitochondrial membrane could well be a relative disorder of structural components initially but controlled by evolutionary forces culminating in an effective alignment providing maximum efficiency. This could well be the arrangement found in the bovine heart mitochondria where a P/O ratio close to three is invariably found. An ordered membrane must be invoked when developing a hypothesis to explain the three coupling sites of the respiratory complexes with the F1F0-ATP synthase. Without convincing evidence of alternatives to date, the honeycomb repeating pattern seems to be the most suitable, if not the only plausible candidate, The high efficiency of any flat honeycomb structure is not in question [55]. All that is required to account for the 3 coupling sites within the plane of the membrane is for the I, III and IV complexes to be arranged around the F1F0- ATPase as follows: the complexes abut on areas of the membrane constituting the low areas, the thinner surrounds of the F1 complex (Figure 2), that can provide the capacitance (condenser) to hold the charge resulting from electron transport along the chain. Each complex will provide charge separation across the coupling membrane: that charged membrane area is approximately one-third of the surface area of the ordered membrane in total. The inner mitochondrial membrane is envisaged as repeating tripartite structures within a predominantly hexagonal arrangement. This is in keeping with the structural studies of Tsuprun et al. [40]. As a consequence of this, when one ET complex (complex I, for example) is inhibited one-third of the membrane capacitor charge is lost and the P/O ratio is reduced by one. When site III is inhibited also, two-thirds of the membrane capacitor charge is lost approximately and so on. This configuration in the coupling membrane is made possible by virtue of molecular chirality and Nesci et al. (2015) provide evidence in support of this such that the chiral α component of the ATP synthase could be orchestrating the asymmetry of other subunits: a phenomenon not uncommon in biological structures [56, 57]. This becomes significant in this discussion and could conceivably have relevance to evolution and the origin of life itself [9]. Apparently, life evolved toward chirality and the mitochondrion could well conform to that pattern. Unfortunately, any link between a confined configuration change and its functionality is not always clear and can lead to intriguing speculations, manifest in this hypothesis [56].
One surprising and incompletely explained deduction based on the symmetries of c-rings in the rotor of the enzyme is that the amount of energy required by the ATP- synthase to make an ATP molecule does not have a universal value [39]. ATP synthases from multicellular organisms require the least energy, whereas the energy required to make an ATP molecule in unicellular organisms and chloroplasts is higher, and a range of values has been calculated. This strongly favours the evolution of oxidative phosphorylation with three neat coupling sites not being de novo and universal but more-so part of a Darwinian process directed towards the highest efficiency. A change of a molecular component and configuration of F1F0-ATPase culminating in the most efficient LDW/HDW could account for this in a manner consistent with the evolution of an enzyme-water mutualism, eventually producing a P/O ratio of approximately three. The model developed by Song et al. (2013) to examine the morphology and PMF of the IMM provides evidence for the inhomogeneity of the IMM and consequently, the resultant concave geometry and cristae folding [58]. According to these authors, proteins, including ATP synthase, are not evenly distributed in the IMM [58]. Variations in the structural organisation of the IMM, in particular the ATP synthetase lends support to the concept of an evolution of the membrane towards higher efficiency, approximating the equivalent of three phosphorylation coupling sites ultimately [59].
Finally, evidence is growing for other roles of ATP synthases in the inner membranes of mitochondria. Here the enzymes form supramolecular complexes, possibly with specific lipids, and these complexes may contribute to, or even determine curvature and the formation of the mitochondrial cristae [39, 60]. There is evidence appearing together with six-fold membrane structures and more infrequently five-fold symmetry units [60, 61, 62]. This variation in the spatial organisation of the constituent structures allows the IMM to fold and cristae to form. Otherwise, an uninterrupted six-fold honeycomb pattern of repeating structures throughout would result in the IMM being a flat sheet, unable to fold and without sidedness providing for matrix and external milieu.
Summary
The object of this hypothesis is to explain oxidative phosphorylation as a three-dimensional arrangement of macromolecular structures with the inner mitochondrial membrane. The redox coupling sites, energised by a change in H₂O-H₂O hydrogen bonding, are juxtaposed in an organised manner around the F1F0-ATP synthase to provide for 3 energy coupling sites along the electron transport chain.
Acknowledgements
The image copyright permissions are solely the responsibility of the authors.
Competing interests
The authors declare no conflict of interest.
Original submitted files for images
Below are the links to the authors’ original submitted files for images.
Original image file for Figure 1
Original image file for Figure 2
Original image file for Figure 3
References
- A possible mechanism for the Na, K-ATPase. J Theor Biol. 1982;Dec 21;99(4):665-79. doi:10.1016/0022-5193(82)90193-x
- Role of water in some biological processes. Microbiol Rev. 1990;Dec;54(4):4-12. doi:10.1128/mr.54.4.432-449.1990
- Rastogi SC Cell and Molecular Biology, 3rd ed. New Delhi, India: New Age International Publishers 2013 - 461 978822430790
- Macromolecular shape and surface maps by solvent exclusion. Proc Natl Acad Sci USA. 1978;Jan;75(1):303-7. doi:10.1073/pnas.75.1.303
- Structure of mitochondrial F1-ATPase studied by electron microscopy and image processing. Biochim Biophys Acta. 1986;Oct 8;851(3):353-60. doi:10.1016/0005-2728(86)90071-x
- Three-dimensional structure of the vacuolar ATPase. Localization of subunit H by difference imaging and chemical cross-linking. J Biol Chem. 2004;Oct 1;279(40):41942-9. doi:10.1074/jbc.M407821200
- The role of aqueous interfaces in the cell. Adv Colloid Interface Sci. 2003;Apr 25;103(2):173-96. doi:10.1016/S0001-8686(02)00095-7
- Water is an active matrix of life for cell and molecular biology. Proc Natl Acad Sci USA. 2017;Dec 19;114(51):13327-35. doi:10.1073/pnas.1703781114
- Life depends upon two kinds of water. PLoS One. 2008;Jan 9;3(1):e1406-. doi:10.1371/journal.pone.0001406
- Ueber die Constitution des flüssigen Wassers [On the constitution of liquid water]. Ann. Phys. 1892;281:91-97. doi:10.1002/andp.18922810108
- A theory of water and ionic solution. With particular reference to the hydrogen and hydroxyl Ions. J. Chem. Phys. 1933;1:515-48. doi:10.1063/1.1749327
- Molecular association in liquids II. A Theory of the structure of water. Proc. R. Soc. London Series A. 1951;205:163-78. doi:10.1098/rspa.1951.0024
- How water's properties are encoded in its molecular structure and energies. Chem. Rev. 2017;117(19):12385-414. doi:10.1021/acs.chemrev.7b00259
- On the existence of two states in liquid water: Impact on biological and nanoscopic systems. Int J. Nanotechnol. 2016;13(8/9):667-. doi:10.1504/IJNT.2016.079670
- The Fourth phase of water. Beyond solid, liquid and vapour. Seattle, USA: Ebner & Sons Publishers. 2013.Chapter 6
- Structures of high-density and low-density water. Phys. Rev. Lett. 2000;84(13):2881-. doi:10.1103/PhysRevLett.84.2881
- Enzyme-water mutualism: implicational speculation. Water. 2019;Jan 17; 10:99-103. doi:10.14294/WATER.2018.8
- A possible role for water in the performance of cellular work: II. Measurements of scattering of light by actomyosin. Bioelectrochem. Bioenerg. 1979; 6(2):135-146. doi:10.1016/0302-4598(79)87003-8
- The hydrophobic effect and the organization of living matter. Science. 1978;Jun 2;200(4345):1012-18. doi:10.1126/science.653353
- The functional role of the hemoglobin-water interface. Mol Aspects Med. 2022;Apr; 84:101042-. doi:10.1016/j.mam.2021.101042
- The nature of the accessible and buried surfaces in proteins. J Mol Biol. 1976;Jul 25;105(1):1-14. doi:10.1016/0022-2836(76)90191-1
- Bioenergetics. New York: Academic Press. 1957;-:36,38,60-.
- Reconstruction of active regular motion in amoeba extract: dynamic cooperation between sol and gel states. PLoS One. 2013;Aug 5;8(8):e70317-. doi:10.1371/journal.pone.0070317
- Actin-based cell motility and cell locomotion. Cell. 1996;Feb 9;84(3):371-9. doi:10.1016/s0092-8674(00)81281-7
- "Squiggle-H2O". An enquiry into the importance of solvation effects in phosphate ester and anhydride reactions. Biochim Biophys Acta. 1970;Nov 3;223(1):1-15. doi:10.1016/0005-2728(70)90126-x
- The source of some of the extraordinary powers and properties of enzymes. Water. 2009;1:35-41. doi:10.14294.WATER.2009.1
- Properties of liquid water: the origin of the density anomalies. J Phys. Chem. 1994;98:2222-2230. doi:10.1021/j100060a002
- Role of hydration water in protein unfolding. Biophys J. 1999;Dec;77(6):3311-8. doi:10.1016/S0006-3495(99)77162-6
- Energetics of potassium transport in mitochondria induced by valinomycin. Biochemistry. 1966;Jul;5(7):2326-35. doi:10.1021/bi00871a022
- Induction of K+ transport and swelling in isolated heart mitochondria by mercurial compounds. Biochem. Biophys. Res. Commun. 1967;Aug 7; 28(3):420-425. doi:10.1016/0006-291X(67)90328-2
- The relationship between the size of mitochondria and the intensity of light that they scatter in different energetic states. Biochimica et Biophysica Acta (BBA). 1981;637(1):146-51. doi:10.1016/0005-2728(81)90220-6
- The ATP synthase--a splendid molecular machine. Annu Rev Biochem. 1997;66:717-49. doi:10.1146/annurev.biochem.66.1.717
- Energy, Life, and ATP (Nobel Lecture). Angew Chem Int Ed Engl. 1998;Sep 18;37(17):2296-2307.
- Insights into the origin of the high energy-conversion efficiency of F1-ATPase. Proc Natl Acad Sci USA. 2019;Aug 6;116(32):15924-15929. doi:10.1073/pnas.1906816116
- Catalytic site forms and controls in ATP synthase catalysis. Biochim Biophys Acta. 2000;May 31;1458(2-3):252-62. doi:10.1016/s0005-2728(00)00077-3
- ATP synthase. Annu Rev Biochem. 2015;84:631-57. doi:10.1146/annurev-biochem-060614-034124
- Coupling of phosphorylation to electron and hydrogen transfer by a chemi-osmotic type of mechanism. Nature. 1961;Jul 8; 191:144-8. doi:10.1038/191144a0
- A protet-based, protonic charge transfer model of energy coupling in oxidative and photosynthetic phosphorylation. Adv Microb Physiol. 2021;71:1-177. doi:10.1016/bs.ampbs.2021.01.001
- The ATP synthase: the understood, the uncertain and the unknown. Biochem Soc Trans. 2013;Feb 1;41(1):1-16. doi:10.1042/BST20110773
- Structure of ATP synthetase studied by electron microscopy and image processing. FEBS Letters. 1989;244(2):279-282. doi:10.1016/0014-5793(89)80545-9
- Proton solvation and transport in aqueous and biomolecular systems: insights from computer simulations. J Phys Chem B. 2007;May 3;111(17):4300-14. doi:10.1021/jp070104x
- Electrostatics, hydration, and proton transfer dynamics in the membrane domain of respiratory complex I. Proc Natl Acad Sci USA. 2014;May 13;111(19):6988-93. doi:10.1073/pnas.1319156111
- Catalytic robustness and torque generation of the F1-ATPase. Biophys Rev. 2017;Mar 25;9(2):103-118. doi:10.1007/s12551-017-0262-x
- Structure and function of mitochondrial membrane protein complexes. BMC Biol. 2015;Oct 29; 13:89-. doi:10.1186/s12915-015-0201-x
- Clarifying the supercomplex: the higher-order organization of the mitochondrial electron transport chain. Nat Struct Mol Biol. 2017;Oct 5;24(10):800-808. doi:10.1038/nsmb.3460
- Rotation and structure of FoF1-ATP synthase. J Biochem. 2011;Jun;149(6):655-64. doi:10.1093/jb/mvr049
- Visualization of the hexagonal lattice in the erythrocyte membrane skeleton. J Cell Biol. 1987;Mar;104(3):527-36. doi:10.1083/jcb.104.3.527
- Correlation between the substructure of the luminal plasma membrane of the bladder in man and other mammals. source. 1972-73;Micron 4 (3):257-267. doi:10.1016/0047-7206(72)90019-2
- Analysis of membrane structure in the transitional epithelium of rat urinary bladder. 2. The discoidal vesicles and Golgi apparatus: their role in luminal membrane biogenesis. J Ultrastruct Res. 1979;Nov;69(2):279-96. doi:10.1016/s0022-5320(79)90117-5
-
Honeycomb structure responsible for bacteria's extraordinary senses.
Cornell News. 2012;Feb. Edition.:---.
Available from: https://phys.org/news/2012-02-honeycomb-responsible-bacteria-extraordinary.html - Specific lipid-protein interactions in a novel honeycomb lattice structure of bacteriorhodopsin. Acta Crystallogr D Biol Crystallogr. 1999;Jul;55(Pt 7):1251-6. doi:s090744499900503x
- Hexagonal lattice of subunits in the thick luminal membrane of the rat urinary bladder. Nature. 1969;Dec 27;224(5226):1304-5. doi:10.1038/2241304a0
- An investigation of mitochondrial inner membranes by rapid-freeze deep-etch techniques. J Cell Biol. 1989;Jun;108(6):2233-40. doi:10.1083/jcb.108.6.2233
- Megacomplex organization of the oxidative phosphorylation system by structural analysis of respiratory supercomplexes from potato. Biochim Biophys Acta. 2009;Jan;1787(1):60-7. doi:10.1016/j.bbabio.2008.10.010
- A proof of the Kepler conjecture. Annals of Mathematics. 2005;162(3):1065-1185. doi:10.4007/annals.2005.162.1065
- Opposite rotation directions in the synthesis and hydrolysis of ATP by the ATP synthase: hints from a subunit asymmetry. J Membr Biol. 2015;Apr;248(2):163-9. doi:10.1007/s00232-014-9760-y
- The origin of chirality in the molecules of life: a revision from awareness to the current theories and perspectives of this unsolved problem. RCS Publishing, Cambridge. 2009.March doi:10.1002/ange.200805910
- Biophysical significance of the inner mitochondrial membrane structure on the electrochemical potential of mitochondria. Phys Rev E Stat Nonlin Soft Matter Phys. 2013;Dec;88(6):062723-. doi:10.1103/PhysRevE.88.062723
- Dimer ribbons of ATP synthase shape the inner mitochondrial membrane. EMBO J. 2008;Apr 9;27(7):1154-60. doi:10.1038/emboj.2008.35
- An electron microscopic demonstration of a surface pattern on the plasma membrane of sectioned intestinal epithelium after saponin treatment. Nature. 1962;Sep 8;195:1023-4. doi:10.1038/1951023b0
- Structure of the ATP-synthase from chloroplasts and mitochondria studied by electron microscopy. Z Naturforsch C J Biosci. 1988;Mar-Apr;43(3-4):219-25. doi:10.1515/znc-1988-3-412
- The ultrastructure of cell membranes and their derivatives. Biochem Soc Symp. 1959.:16-45.