In vitropre-vascularisation of tissue-engineered constructs A co-culture perspective
Vascular Cell. 2014;
Received: 16 February 2014 | Accepted: 12 June 2014 | Published: 21 June 2014
Vascular Cell ISSN: 2045-824X
Abstract
Keywords
Co-culture Vascularisation Tissue engineering MatricesIntroduction
Researchers have two main options when vascularizing tissue-engineered constructs: either implant the construct
Cell source
A key first decision in designing an
Endothelial and precursor cells
Endothelial cells are present in most tissues within the human body; however, their relative abundance and composition varies from tissue to tissue [12]. A microarray study on the expression profiles of 53 endothelial cells showed distinct tissue-specific expression patterns in cells isolated from different blood vessels and microvasculature in the body [13]. There are a wide variety of different types of endothelial cells used in the literature. Researchers seeking to model a particular biological system or disease state may choose to isolate them directly from the tissue of interest. The logic behind isolating cells from the tissue of interest is that the researchers will be able to isolate endothelial subpopulations specific to the microenvironment that they wish to recapitulate. However, from a tissue engineering perspective, isolating tissue-specific endothelial cells may not be a feasible strategy as retrieving these cells may require an invasive procedure, and in the case of major organs or tissues may not be a viable option. In order for a specific cell-based tissue engineering approach to be practical in a clinical setting, the source of cells needs to be (i) relatively abundant, (ii) readily available and (iii) pose a minimal to low risk to patient/donors. Examples of non-invasive cell sources include placental or umbilical cords which are commonly discarded as medical waste, and examples of minimally invasive procedures for isolation of endothelial cells include peripheral blood and skin biopsy [14–16].
It is important to remember that isolated primary cells are heterogeneous and contain a mix of different endothelial cell subpopulations. In 2004 Ingram et al. identified a novel cell hierarchy among endothelial cells found in human peripheral and umbilical cord blood based on clonogenic and proliferative potential [17]. The endothelial lineage is believed to follow a similar hierarchical as myeloid and lymphoid lineages in which a primitive stem cell gives rise to proliferating progenitor cells, followed by the progression to terminally differentiated cells [17]. Figure 1 shows the model of endothelial cell hierarchy based on proliferative and clonogenic potential, thus defining endothelial progenitors (EPC) as cells giving rise to high proliferative colonies with the capacity to form blood vessels upon transplantation. A further study identified a subpopulation of endothelial progenitor cells (EPC) within human umbilical vein endothelial cells (HUVEC) and human aortic endothelial cells (HAEC) [18]. Both HUVEC and HAEC can be isolated from vessel walls and were previously thought to consist of only mature differentiated endothelial cells [19]. The heterogeneous composition of isolated endothelial cells may affect the reproducibility of cell-based treatments and isolated cells may need to be sorted into individual cell populations.
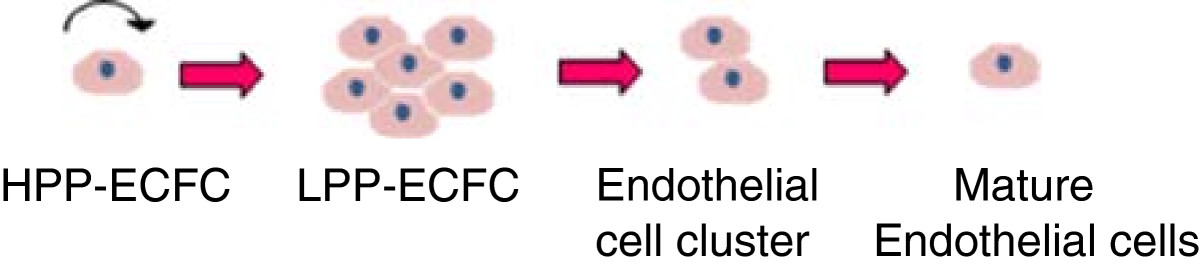
Figure 1
Figure 1 caption
Overview of endothelial cell hierarchy. High proliferative potential- endothelial colony forming cells (HPP-ECFC) give rise to all other subsequent endothelial progenitor cells, can achieve greater than 100 population doublings and can form secondary and tertiary colonies upon replating. Low proliferative potential –endothelial colony forming cells (LPP-ECFC) can give rise to colonies containing more than 50 cells, whilst endothelial cell clusters give rise to colonies with fewer than 50 cells. Neither LPP-ECFC or endothelial clusters can give rise to secondary or tertiary colonies. Mature endothelial cells are terminally differentiated and have a limited proliferative potential [].
The therapeutic potential of EPC subpopulations of endothelial cells has garnered a significant amount of interest within the research community in recent years. EPCs have been shown to have enhanced proliferative potential, are able to differentiate and give rise to all subsets of the endothelial cell lineage and have been shown to improve vasculogenic activity [20]. Other studies have also shown that EPC have a higher survival rate
CFU-Hill are non-adherent PB-MNC that give rise to colonies after 5 days following depletion of adherent cells on fibronectin and CAC cells are adherent PB-MNC that attach to fibronectin or gelatin surface after 4–6 days of culture [24, 25]. Both CFU-Hill and CAC cells co-express CD31, VEGFR-2 and CD133 [27]. CD133 is a hematopoietic stem cell (HSC) marker that is lost as cell differentiate [28]. Peichev et al. hypothesized that CD133 may also be a marker for immature EPC populations [29]. A later study by Case et al. in 2007 however showed that 99% of CD34+ VEGFR-2+ CD133+ cells also co-expressed CD45+, a common leukocyte antigen, not expressed by endothelial cells [30]. In addition CD34+ VEGFR-2+ CD133+ cells also readily ingest bacteria and lacked the ability to form human vessels de novo
ECFC on the other hand are late outgrowth cells that form colonies on type 1 rat tail collagen following 14–21 days of culture [26]. According to Ingram et al. ECFC more closely match the criteria of being a true EPC [17]. Unlike CFU-Hill and CAC cells, ECFCs do not express haemopoetic markers such as CD133 and CD45 [31]. ECFC are highly proliferative and have the potential to form both secondary and tertiary colonies on replating [17]. ECFC are also capable of forming human vessels de novo
The findings from these studies highlight the striking differences between the different classes of putative EPCs and have important implication for their therapeutic application. Although CD34+ VEGFR-2+ CD133+ cells cannot initiate vessel formation de novo
Another major limitation of ECFCs is their rarity within the human body. EPC only make up 0.01% of circulating mononuclear cells in peripheral blood [33]. An alternative source of ECFC is umbilical cord blood or placental tissue. The number of endothelial cell colonies derived from umbilical cord blood was 15 times higher than from equivalent volumes (20 mL) of peripheral blood [17]. The colonies formed by umbilical cord blood were also consistently larger than those isolated from peripheral blood [17]. More recently, it has now become possible to also isolate ECFCs from the placenta tissue. From a single placenta (500-600 g) it is possible to isolated the same amount of ECFCs as found in 27 whole cord blood (60 mL) samples [34]. Gene expression and functional studies have demonstrated that these cells are equivalent to umbilical cord blood derived ECFCs [34]. The high number of ECFCs that can be derived from these tissues, that are commonly discarded, is opening new possibilities in tissue engineering, in particular in large defect applications that require high cell numbers.
An alternative strategy may be to expand isolated ECFC
Mature endothelial cells and endothelial cell clusters lack the ability to be expanded out into high numbers like their ECFC counterparts, however these endothelial cells are still capable of forming capillary-like structures. ECFC cells are responsive to angiogenic factors, have a high survival rate and are believed to play a key role in maintaining vessel wall integrity [18, 19]. Therefore the use of mature endothelial cells/endothelial cluster subpopulations to form an
Multipotent adult stem cells
Adult stem cells are multipotent cells that are capable of differentiating into a narrow range of different cell types [37]. Recent advances in our understanding of stem cell biology and regulation have provided researchers with a range of novel tools and research strategies to guide cell fate both within and outside their traditional cell lineages. Bone marrow mesenchymal stem cells (BM-MSC) have been shown to readily differentiate into endothelial cells under angiogenic conditions. Differentiated BM-MSC express several endothelial markers
Pluripotent stem cells
In the past ten years there has been significant advancement in the fields of stem cell biology and iPS technology [44]. These developments have had a tremendous impact on regenerative medicine and tissue engineering concepts. Unlike endothelial progenitor cells, pluripotent stem cells have the potential of differentiating into all three germ layers [45]. The most recognizable and well characterized pluripotent cells are ESCs. A number of studies have been able to differentiate ESC into endothelial and associated mural cells [46, 47]. ESC-derived endothelial cells were shown to contribute to the construction of new blood vessels and improved blood flow in a hindlimb ischemia model [48]. However ethical concerns surrounding the isolation of ESC limit the widespread application and adoption of this cell technology [49]. Other technical limitations associated with the use of ESC include source availability, difficulty in separating out endothelial cells from undifferentiated ESC cell and the potential for ESC to form teratomas [49–51].
iPS cells are differentiated cells that have been genetically reprogrammed to return to a pluripotent stem cell state and therefore circumvent the need to source cells from embryos [52]. A large body of research from the mid 1990s to early 2000s identified a number of key genes relating to maintenance and regulation of pluripotency in embryos and ESC [53–58]. In 2006 a seminal paper by Dr Shinya Yamanaka’s laboratory at Kyoto University screened 24 of these genes as candidates for inducing pluripotency in somatic cells and identified four factors, including Oct3/4, Sox2, c-Myc and Klf4, that were able to successfully produce iPS cells from mouse adult fibroblast cells [59]. Since this study, a number of laboratories have been able to use Dr. Yamanaka’s technique to generate iPS cells from a variety of different cell types and species including humans [60]. Once the cells have returned to a pluripotent state the cells can then be re-differentiated into endothelial and associated mural cells. Choi et al. were able to differentiate seven human iPS cell lines into endothelial (CD31+, CD34-) cells [61]. The iPS-derived endothelial cells were shown to successfully form capillary-like structures on growth factor reduced matrigel in 2D. Another study by Samuel et al. was able to establish functional blood vessels long-term (280 days)
Pluripotent stem cells represent a potential universal cell source for endothelial cells, but the technology is still in the early stages of development and if researchers cannot rectify the issues associated with the cells, in particular the safety concerns, the technology will never be able to move beyond pre-clinical applications into clinical regenerative therapies.
Supporting cells
Cells that are grown in conjunction with endothelial cells can have both a direct and indirect impact on the development of vascular networks in tissue-engineered constructs. Figure 2 highlights the role that these cells can have on capillary formation and maturation.
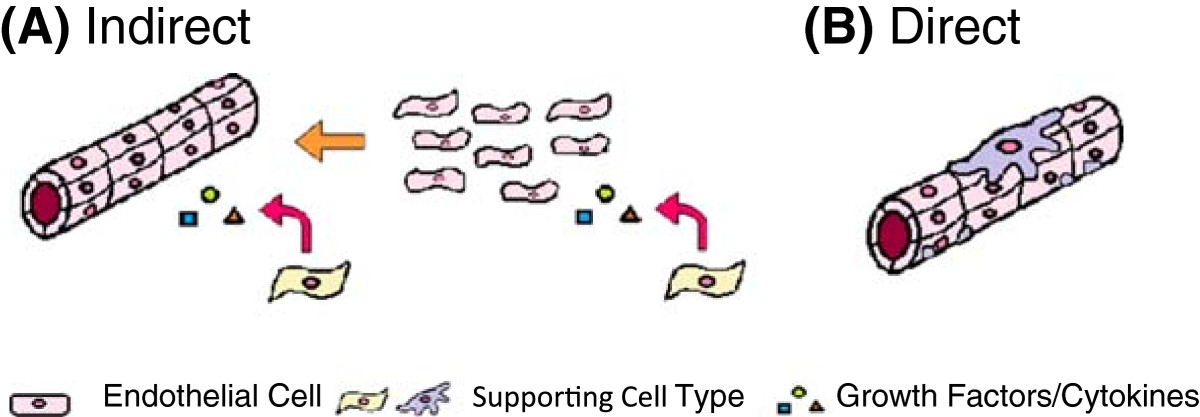
Figure 2
Figure 2 caption
Overview of the roles of supporting cells in capillary formation. (A) Indirect role of supporting cells in establishment and maintenance of capillary structures through release of cytokines and growth factors and (B) direct role of supporting cells in the structural and functional support of blood vessels and capillaries.
Assays for the functional assessment of vascularization
A number of
Although
Co-culture parameters
There are a number of parameters that require careful consideration when designing a co-culture system. Some conditions may be considered trivial, but can have important implications for the end tissue-engineered product.
Scaffold/Matrix selection
In tissue engineering, scaffolds and matrices provide cells with support and structure to move from 2D tissue culture plate into a 3D microenvironment. The three main types of 3D scaffolds include; solid scaffolds, hydrogels (Figure 3A-B) or a combination of the two constructs (Figure 3C). Solid scaffolds are porous 3D structures, whilst gels are polymer networks that are expanded throughout their volume by fluid. Thus far, only hydrogels have been shown to form functional vascular networks with lumen
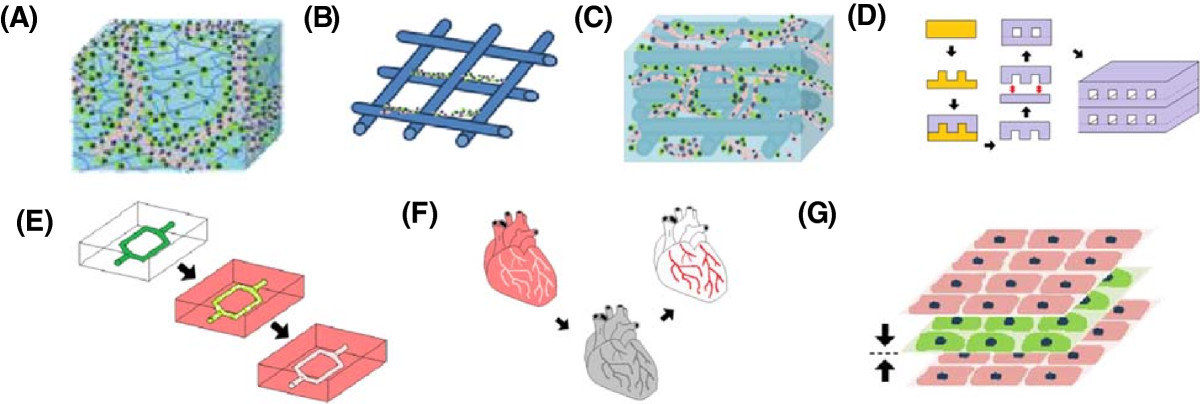
Figure 3
Figure 3 caption
Overview of tissue engineering constructs. Endothelial cells encapsulated within a (A) hydrogel and (B) seeded on a solid scaffold. Only functional capillaries with lumen form in hydrogels. (C) Hybrid construct combining the tube forming capabilities of the hydrogel with the structural support of a scaffold. (D) Photolithography used to etch microchannels into a silicon master mold. Biomaterial cast into mold to create a patterned surface. Patterned layer bonded to flat unpatterned surface to create closed system. Multiple layers can be fused together to create larger 3D constructs. (E) Biodegradable material dissolves in biomaterial to leave patterned microchannels. (F) Whole tissue organs decellularized leaving the ECM intact with a hollow vasculature network that can later be re-seeded with endothelial cells. (G) Cell sheeting engineering used to create vascularized 3D constructs by sandwiching endothelial cell sheets with non-endothelial cell sheets together.
However, it is important to note the immersion of cells in a 3D environment by itself also will not lead to capillary formation
The mechanical properties of a construct can also play an important role in capillary formation. In literature the range of stiffness as measured by compression modulus for optimal tube formation in hydrogels varies between different gel types, however in all cases increased vasculature formation was associated with decreasing stiffness [92–95]. The phenotypic expression of other cell types on the other hand can increase with increasing stiffness. For example, in bone tissue engineering, stiffer gels can increase bone mineralization and expression of differentiation markers such as osteocalcin, osteopontin and alkaline phosphatase [96, 97]. The selection of optimal construct stiffness can be problematic if attributes (i.e. vascularisation and bone formation) are desired in the same construct, but inversely related. Researchers need to find a middle ground or alter another parameter in the system.
Fabrication of microchannels
A new wave of vascular constructs and designs are helping to speed up the tube formation process and assist with co-culture strategies. The process of capillary formation
Concepts from microfluidics can be utilized to engineer vasculature for tissue engineering. Microfabrication techniques, such as photolithography, can be used to etch micron sized open channels into a silicon master mould (Figure 3D) [100]. A polymer of interest can then be poured into the mould in order to create a patterned surface. Once set the patterned layer can be removed and then bonded with a flat unpatterned layer of polymer to create a closed system. Different polymer layers can be fused using plasma treatment, temperature/pressure difference, or other polymer specific properties. These microfluidic constructs can then be further stacked and bonded to create larger 3D tissue engineered constructs. A major limitation of the layer bonding technique is the danger of leaks if the layers are not completely fused together.
Another microfluidic approach is to mould a biodegradable material into the shape of a vasculature network and then embed the construct within a biomaterial of interest (Figure 3E). The construct acts as a sacrificial material that degrades over time to leave hollow microchannels behind. This method can also be combined with additive manufacturing techniques to create complex 3D vasculature structures [101]. Another benefit of biodegradable microfluidic channels is that unlike the layer bonding method the surrounding biomaterial formed is intact and there is a lower danger of leaks forming, however the drawback is that the technique doesn’t have the high resolution of other microfabrication techniques such as photolithography.
A final approach is to re-endothelialise decellularized whole tissue or organs using the existing vessel structures as templates (Figure 3F). Advances in decellularization processes in recent years have now made it possible to remove cells from tissue whilst retain the vital structure and bioactivity of the ECM [102]. The organs and tissue can also be sourced from xenogenic tissues which are readily available. A limitation of this approach is that users are restricted to the layout of vascular structures in the tissue. There are also still unresolved concerns surrounding the antiginicity, immunogenicity and shelf life of decellularized organs [103].
Cell sheet technology
A final technique for developing tissue engineered constructs is cell sheet technology (Figure 3G). Cell sheet engineering is a non-scaffold based approach that uses temperature responsive cell culture surfaces to harvest intact cell sheets that can be stacked together to reconstructs 3D tissue [104]. The temperature responsive culture surfaces are created by treating normal tissue culture plates with poly(N-isoproplyacrylamide)(PIPAAm) that can alternate between states of hydrophobicity and hydrophilicity [105]. At temperatures higher than 32 degrees the substrate is hydrophobic and cells can attach to the surface and form a confluent layer. Lowering the temperature below 32 degrees causes the substrate to become hydrophilic and the cells sheet and ECM to detach from the surface. A gel coated plunger can then be used to manipulate and stack the cell sheets. The cell sheet technique has been used to effectively replicate tissue and organs, such as skin and cardiac tissue, both
Cell ratio
The ratio between the different cell types in co-culture can influence cell characteristics, behavior and survival. In view of the available literature, no consensus exists on the optimal cell ratio of endothelial cells to tissue-specific cells for use in
A key characteristics to consider when selecting the ratio of cells to use in a co-culture system is the cells individual metabolic and proliferative potential. If the cells proliferative and metabolic activity differs significantly, depending on the duration of
Culture medium
The composition of culture media is one of the key points to consider when culturing cells
The use of serum is another important consideration in endothelial cell culture. Serum is a common media additive to support cell growth and viability
In a co-culture scenario each cell will have its own individual media requirements. In some cases the cells may utilise the same media, but often this will not be the case. If cells require different culture media, researchers need to optimize an appropriate media combination that offers acceptable viability, whilst promoting or maintaining desired cell phenotype. Most papers do not explain the decisions that led them to the selection of their chosen media or media combination, despite the critical role that this factor may play in the outcome of the study. The media mixture may depend on the ratio of each cell type used, the sensitivity of cells - one cell type being potentially more sensitive than another to alteration of media composition - and the purpose of the study.
The addition of supplements to media is another issue that has to be considered. Co-culturing cells together will change the expression profile of each cell type through paracrine signaling and cell-cell interactions. The endogenous factors secreted by the cells in the microenvironment may contribute to, or may inhibit, the usual effect of supplements in the medium. For example, Unger et al. showed that in monoculture of HDMEC, exogenous angiogenic factors, such as bFGF or VEGF, were required for microvascular formation [68]. However, surprisingly when these components were added to co-cultures of HDMEC and osteoblasts, microcapillary formation did not occur. VEGF promotes endothelial cell motility, and in this case too much of this factor may be over stimulating the cells and destabilizing the network.
There may also be a potential significant difference between endothelial capillary formation that is driven by exogenous stimulus in the form of angiogenic supplements added to culture media versus endogenous angiogenic factors secreted by non-endothelial supporter cells in a co-culture system. The exogenous supplements are added as a single dose at the time of media change and their bioactivity will decrease with time depending on the initial concentration, stability of the supplement and relative uptake by the cells. Factors secreted by supporting cells in co-culture are released more steadily over time but as with the exogenous supplements the factors will be removed when the media is changed. Figure 4 shows a visual representation of this phenomenon. It is unclear how the difference between exogenous and endogenous stimulated capillary formation may influences the structural and functional aspects of the networks. In the end, the best way to determine the optimal medium composition may be experimentally by examining not only the proliferation or viability of each cell type but its impact on gene expression and cell phenotype.
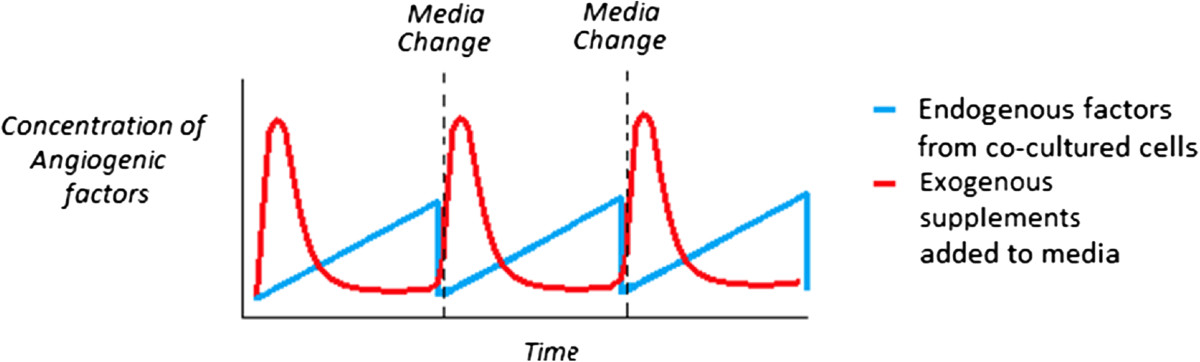
Figure 4
Figure 4 caption
Representation of the difference between angiogenic factors supplied by endogenous support cells in co-culture versus the use of exogenous supplements to media in a static system. Angiogenic factors from an exogenous source (red) are introduced into the system in a spike dependent manner and reduce over time, whilst endogenous angiogenic factors (blue) are released into the system steadily over time.
Seeding technique
There are two main types of seeding parameters researchers can modulate: temporal (seed simultaneously or sequentially) and spatial (seed on one construct or multiple constructs).
Seeding the cells in the matrix at the same time allows for a homogeneous mix of cells throughout the construct (Figure 5A). This is beneficial if cell-cell contact is important for cell function or if the cell types are naturally co-located with one another in the tissue of interest. Researchers can also use the same scaffold, but seed the cells at different times (Figure 5B). In addition to modifying the cell ratio, sequential seeding is beneficial if the cell proliferation rates differ significantly and there is the potential that the more proliferative cell type may take over the construct. Moreover, pre-seeding one cell type in the scaffold may help direct or bias the overall characteristics of the construct towards a particular phenotype or trait of interest. For example, Lyer et al. previously showed that following co-culture of EC, fibroblasts and cardiomyocytes in matrigel, the cells formed an organoid that mimicked cardiac structure and function, but the EC cells did not organize into capillary structures [131]. However, a separate study from the same group seeded the EC cells first, followed by fibroblasts 24 hours later, and cardiomyocytes 48 hours later, which resulted in extensive cord formation in the end construct [132]. Seeding the EC cells first may have provided the cells with time to form tubes unimpeded and the addition of fibroblasts may have provide the newly formed network with structural support before the addition of the cardiomyocytes. A difficulty associated with sequential seeding in the same construct is the requirement to incorporate cells in a solid scaffold or gel that has already been made. The construct would need to be either porous, include hollow microchannels, or require a chemoattractant to encourage cell ingrowth. In the case of Lyer et al., the organoids were thin microtissue [132].
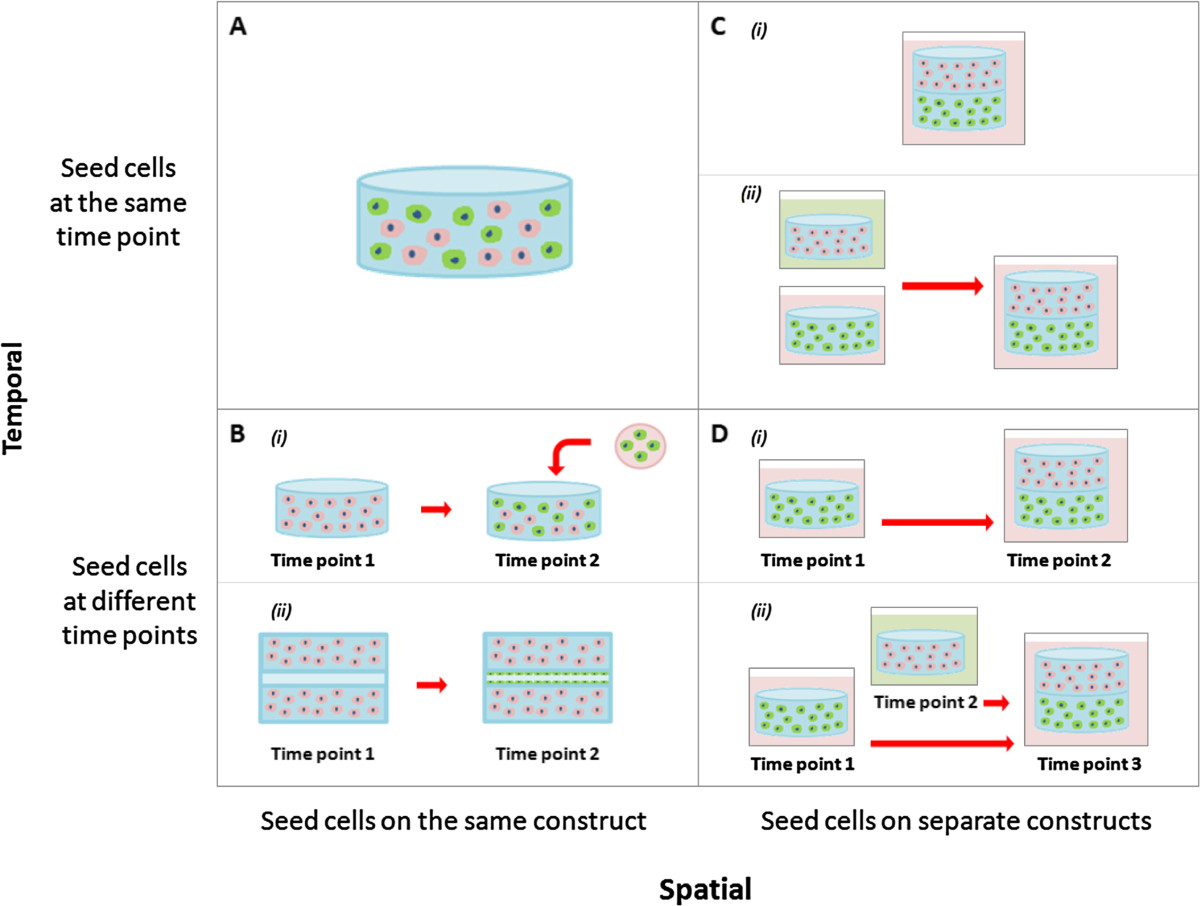
Figure 5
Figure 5 caption
Different cell seeding strategies for co-culture systems in tissue engineering. (A) Cells seeded together in the same construct at the same time point. (B) Cells seeded together in the same construct at different time points. (C) Cells seeded in different constructs at the same start point and either cultured (i) together or (ii) separately. (D) Cells seeded in different constructs at different time points and either cultured (i) together or (ii) separately.
Cells can also be spatially separated on different scaffolds (Figure 5C-D). As previously mentioned, the properties of scaffolds can influence the phenotype of the cells in the tissue-engineered constructs, which may be problematic if competing characteristics are required. A way around this problem is to place the cells in separate scaffolds with optimal properties for each desired cell trait. These scaffolds can be seeded either simultaneously or sequentially but again will depend on the proliferation rates of the cells and the desired cell traits in the construct. An added advantage of spatially separated constructs is that they can also be cultured in different medias before being combined (Figure 5Cii-Dii). This can overcome some of the possible problems associated with compromising on shared media conditions. The limitation of spatially separating the cells is that it allows only minimal cell-cell contact between the different cell types.
Dynamic systems
Bioreactor systems are commonly used within an
It has been long known that hypoxia can promote angiogenesis and vascularisation in tissue [134]. An oxygen dependent homeostatic mechanism in the body ensures that all cells receive adequate blood supply [135]. When tissues are exposed to a low oxygen environment they begin to express factors such as hypoxia-inducible factor 1(HIF-1) which promotes VEGF production [135]. The VEGF then acts on the endothelial cells to promote cell migration and vascularisation. Researchers can mimic this cellular response by modulating oxygen tension in a controlled environment such as a incubator to control capillary formation in endothelial cells
Studies relating to the utilization of rotational bioreactors in co-culture systems have so far shown mixed results. Xing et al. immobilized scaffolds co-cultured with bone marrow stromal cells and endothelial cells on stationary needles in a spinner flask moving in a single direction on the x-axis [140]. After a week in the bioreactor, extensive capillary networks formed within the scaffolds. However, in a study by Liu et al. that co-cultured EPC and MSC on immobilized scaffolds using a biaxial bioreactor which was rotating simultaneously on a perpendicular axes (X and Z), no vessel formation was observed in the dynamic system, but extensive vessel network formed under static conditions after a week of culture
Perfusion systems can be used to mimic the haemodynamic forces and pressures that occur naturally in the human body. Fluid flow in a bioreactor can be directed through the bulk of a construct, however in most cases it will be directed through hollow microchannels or pores within the construct, similar to those described in section 3.1, that have been pre-seeded with endothelial or perivascular cells. Several studies have shown that mechanical stimulation under peristaltic flow conditions can increase the production of ECM proteins, such as elastin and collagen, and improve the mechanical properties of the vessel or capillary as measured by burst pressure and resistance to shear stress [142]. Mechanical stimulation by perfusion systems is critical in pre-conditioning vascular constructs prior to implantation.
Conclusion
There are a variety of cell sources for vascularized tissue constructs; however, endothelial cells are the one cell type that is ubiquitous in almost all systems. EC are heterogeneous in nature and contain a mix of subpopulations. EPC cells hold great promise in the field and have been shown to enhance proliferative ability, survival rate and angiogenic potential. Stem cell-derived EC also represent a viable alternative to directly isolating endothelial cells and its precursors, however issues including ethical concerns, source availability and tumourgenicity limit their application. Other cell types co-cultured with endothelial cells have also been shown to play both direct and indirect roles in the development and maturation of capillary networks.
The selection of appropriate scaffolds is also an important consideration. ECs require a 3D environment, with adhesion and degradation sites, in order to form functional tube structures with a lumen. EC capillary formation is also strongly associated with decreasing hydrogel stiffness. Modifying cell ratios can help prevent one cell type taking over the construct and/or push a co-culture system towards a particular desired cell trait. When optimizing cell culture media, researchers need to take into account the factors released by both cell types as it changes the dynamics of the culture. Supplements that previously supported a cell type in monoculture may not be required or may even have a detrimental effect on cells in co-culture. Finally, specialized seeding techniques and dynamic bioreactors can be used to overcome barriers in co-culture systems, but the optimal strategy will depend on the desired outcome.
Balancing all these conditions can be difficult, and with increasing number of novel biomaterials, cell isolation strategies, media formulations, seeding techniques and bioreactor systems being developed, the variety of options available to researchers is only set to continue. However, it is important for researchers to be able to identify parameters, understand the interrelationship between variables and appreciate the knock-on effect that changing of different conditions can have on a co-culture system, in order to help them appropriately design their experiments and achieve the desired research outcomes.
Additional files
Additional file 1: Table S1: Summary of various growth factors and cytokines secreted by support cells to promote capillary formation, stabilization and maturation [143–153]. (DOCX 13 KB)
Acknowledgment
K Khosrotehrani was supported by the NHMRC Australia (project grant 1023368 and fellowship 1023371).
Authors’ original submitted files for images
Below are the links to the authors’ original submitted files for images.
Authors’ original file for figure 1
Authors’ original file for figure 2
Authors’ original file for figure 3
Authors’ original file for figure 4
Authors’ original file for figure 5
Authors’ original file for figure 6
References
- Vascularization in tissue engineering. Trends Biotechnol. 2008;26:434-441.
- Engineering thick tissues—the vascularisation problem. Eur Cell Mater. 2007;14:1-18.
- Co-culture systems for vascularization—learning from nature. Adv Drug Deliv Rev. 2011;63:291-299.
- Hyperoxia, endothelial progenitor cell mobilization, and diabetic wound healing. Antioxid Redox Signal. 2008;10:1869-1882.
- CD34+ blood cells accelerate vascularization and healing of diabetic mouse skin wounds. J Vasc Res. 2003;40:368-377.
- Endothelial progenitor cells in cardiovascular disorders. J Am Coll Cardiol. 2007;49:741-752.
- Fracture induced mobilization and incorporation of bone marrow‒derived endothelial progenitor cells for bone healing. J Cell Physiol. 2008;215:234-242.
- Contribution of outgrowth endothelial cells from human peripheral blood on in vivo vascularization of bone tissue engineered constructs based on starch polycaprolactone scaffolds. Biomaterials. 2009;30:526-534.
- Endothelial cell coculture within tissue-engineered cardiomyocyte sheets enhances neovascularization and improves cardiac function of ischemic hearts. Circulation. 2008;118:S145-S152.
- Human dermal microvascular endothelial cells form vascular analogs in cultured skin substitutes after grafting to athymic mice. FASEB J. 2002;16:797-804.
- Endothelial progenitor cells and mesenchymal stem cells seeded onto β-TCP granules enhance early vascularization and bone healing in a critical-sized bone defect in rats. Tissue Eng. 2010;16:1961-1970.
- . Fundamentals of Tissue Engineering and Regenerative Medicine. 2009.
- Endothelial cell diversity revealed by global expression profiling. Proc Natl Acad Sci U S A. 2003;100:10623-10628.
- Isolation of endothelial cells and their progenitor cells from human peripheral blood. Eur J Vasc Surg. 2000;31:181-189.
- Culture of human endothelial cells derived from umbilical veins. Identification by morphologic and immunologic criteria. J Clin Invest. 1973;52:2745-.
- Isolation of endothelial cells from human term placental villi using immunomagnetic beads. Placenta. 1994;15:355-364.
- Identification of a novel hierarchy of endothelial progenitor cells using human peripheral and umbilical cord blood. Blood. 2004;104:2752-2760.
- Vessel wall–derived endothelial cells rapidly proliferate because they contain a complete hierarchy of endothelial progenitor cells. Blood. 2005;105:2783-2786.
- Human endothelial cells derived from circulating progenitors display specific functional properties compared with mature vessel wall endothelial cells. Blood. 2004;103:2577-2584.
- Endothelial progenitor cells and their potential therapeutic applications. Regen Med. 2008;3:863-876.
- In vitro angiogenesis properties of endothelial progenitor cells: a promising tool for vascularization of ex vivo engineered tissues. Tissue Eng. 2007;13:1413-1420.
- Redefining endothelial progenitor cells via clonal analysis and hematopoietic stem/progenitor cell principals. Blood. 2007;109:1801-1809.
- Unresolved questions, changing definitions, and novel paradigms for defining endothelial progenitor cells. Blood. 2005;106:1525-1531.
- Circulating endothelial progenitor cells, vascular function, and cardiovascular risk. N Engl J Med. 2003;348:593-600.
- Number and migratory activity of circulating endothelial progenitor cells inversely correlate with risk factors for coronary artery disease. Circ Res. 2001;89:e1-e7.
- Origins of circulating endothelial cells and endothelial outgrowth from blood. J Clin Invest. 2000;105:71-77.
- Assessing identity, phenotype, and fate of endothelial progenitor cells. Arterioscler Thromb Vasc Biol. 2008;28:1584-1595.
- Biology and plasticity of CD133+ hematopoietic stem cells. Ann N Y Acad Sci. 2003;996:141-151.
- Expression of VEGFR-2 and AC133 by circulating human CD34+ cells identifies a population of functional endothelial precursors. Blood. 2000;95:952-958.
- Human CD34þAC133þVEGFR-2þ cells are not endothelial progenitor cells but distinct, primitive hematopoietic progenitors. Exp Hematol. 2007;35:1109-1118.
- Late outgrowth endothelial cells resemble mature endothelial cells and are not derived from bone marrow. Stem Cells. 2013;31:338-348.
- Strikingly Different Angiogenic Properties of Endothelial Progenitor Cell SubpopulationsInsights From a Novel Human Angiogenesis Assay. J Am Coll Cardiol. 2008;51:660-668.
- Detection of circulating endothelial cells and endothelial progenitor cells by flow cytometry. Cytometry B Clin Cytom. 2005;64:1-8.
- Prospective surface marker-based isolation and expansion of fetal endothelial colony-forming cells from human term placenta. Stem Cells Trans Med. 2013;2:839-847.
- Ex vivo expansion of umbilical cord blood stem cells for transplantation: growing knowledge from the hematopoietic niche. Bone Marrow Transplant. 2007;39:11-23.
- Clinical scale ex vivo expansion of cord blood–derived outgrowth endothelial progenitor cells is associated with high incidence of karyotype aberrations. Exp Hematol. 2008;36:340-349.
- Plasticity of adult stem cells. Cell. 2004;116:639-648.
- Mesenchymal stem cells can be differentiated into endothelial cells in vitro. Stem Cells. 2004;22:377-384.
- Endothelial differentiation of mesenchymal stromal cells. PLoS One. 2012;7:e46842-.
- Human adipose tissue-derived stem cells differentiate into endothelial cells in vitro and improve postnatal neovascularization in vivo. Biochem Biophys Res Commun. 2005;332:370-379.
- Urine derived cells are a potential source for urological tissue reconstruction. J Urol. 2008;180:2226-2233.
- Urine-Derived Stem Cells: Biological Characterization and Potential Clinical Applications. Stem Cells: Current Challenges and New Directions. 2013.:19-28.
- Multipotential differentiation of human urine‒derived stem cells: Potential for therapeutic applications in urology. Stem Cells. 2013;31:1840-1856.
- . Principles of Tissue Engineering. 2013.
- Totipotency, pluripotency and nuclear reprogramming. Engineering of stem cells. 2009.:185-199.
- Derivation of endothelial cells from human embryonic stem cells by directed differentiation analysis of microRNA and angiogenesis in vitro and in vivo. Arterioscler Thromb Vasc Biol. 2010;30:1389-1397.
- Endothelial cells derived from human embryonic stem cells. Proc Natl Acad Sci. 2002;99:4391-4396.
- Pathway for differentiation of human embryonic stem cells to vascular cell components and their potential for vascular regeneration. Arterioscler Thromb Vasc Biol. 2007;27:2127-2134.
- . The human embryonic stem cell debate: science, ethics and public policy. 2001.
- Embryonic stem cell-derived endothelial cells may lack complete functional maturation in vitro. J Vasc Res. 2006;43:411-421.
- Teratoma formation by human embryonic stem cells: evaluation of essential parameters for future safety studies. Stem Cell Res. 2009;2:198-210.
- . Induced Pluripotent Stem Cells. 2012.
- STAT3 activation is sufficient to maintain an undifferentiated state of mouse embryonic stem cells. EMBO J. 1999;18:4261-4269.
- The homeoprotein Nanog is required for maintenance of pluripotency in mouse epiblast and ES cells. Cell. 2003;113:631-642.
- Multipotent cell lineages in early mouse development depend on SOX2 function. Genes Dev. 2003;17:126-140.
- Quantitative expression of Oct-3/4 defines differentiation, dedifferentiation or self-renewal of ES cells. Nat Genet. 2000;24:372-376.
- LIF/STAT3 controls ES cell self-renewal and pluripotency by a Myc-dependent mechanism. Development. 2005;132:885-896.
- A core Klf circuitry regulates self-renewal of embryonic stem cells. Nat Cell Biol. 2008;10:353-360.
- Induction of pluripotent stem cells from mouse embryonic and adult fibroblast cultures by defined factors. Cell. 2006;126:663-676.
- Induced pluripotency: history, mechanisms, and applications. Genes Dev. 2010;24:2239-2263.
- Hematopoietic and endothelial differentiation of human induced pluripotent stem cells. Stem Cells. 2009;27:559-567.
- Generation of functionally competent and durable engineered blood vessels from human induced pluripotent stem cells. Proc Natl Acad Sci. 2013;110:12774-12779.
- Epigenetic memory in induced pluripotent stem cells. Nature. 2010;467:285-290.
- The promise of human induced pluripotent stem cells for research and therapy. Nat Rev Mol Cell Biol. 2008;9:725-729.
- Mural cell associated VEGF is required for organotypic vessel formation. PLoS One. 2009;4:e5798-.
- The effect of human osteoblasts on proliferation and neo-vessel formation of human umbilical vein endothelial cells in a long-term 3D co-culture on polyurethane scaffolds. Biomaterials. 2008;29:4217-4226.
- Induction of tube formation by angiopoietin‒1 in endothelial cell/fibroblast co‒culture is dependent on endogenous VEGF. Cancer Sci. 2003;94:782-790.
- Tissue-like self-assembly in cocultures of endothelial cells and osteoblasts and the formation of microcapillary-like structures on three-dimensional porous biomaterials. Biomaterials. 2007;28:3965-3976.
- Crosstalk between osteoblasts and endothelial cells co-cultured on a polycaprolactone–starch scaffold and the in vitro development of vascularization. Biomaterials. 2009;30:4407-4415.
- Chondrocytes inhibit endothelial sprout formation in vitro: Evidence for involvement of a transforming growth factor‒beta. J Cell Physiol. 1991;146:170-179.
- Inhibition of tubular morphogenesis in human microvascular endothelial cells by co-culture with chondrocytes and involvement of transforming growth factor β: a model for avascularity in human cartilage. Biochimica et Biophysica Acta (BBA)-General Subjects. 1994;1201:135-142.
- Inhibition of vascular endothelial cell morphogenesis in cultures by limbal epithelial cells. Invest Ophthalmol Vis Sci. 1999;40:1822-1828.
- . Human Physiology: From Cells to Systems. 2011.
- Molecular regulation of vessel maturation. Nat Med. 2003;9:685-693.
- Molecular basis of endothelial cell morphogenesis in three‒dimensional extracellular matrices. Anat Rec. 2002;268:252-275.
- Complexity in biomaterials for tissue engineering. Nat Mater. 2009;8:457-470.
- Coregulation of vascular tube stabilization by endothelial cell TIMP-2 and pericyte TIMP-3. J Cell Biol. 2006;175:179-191.
- Endothelial cell-pericyte interactions stimulate basement membrane matrix assembly: influence on vascular tube remodeling, maturation, and stabilization. Microsc Microanal. 2012;18:68-80.
- Human Endothelial Progenitor Cells Induce Extracellular Signal-Regulated Kinase-Dependent Differentiation of Mesenchymal Stem Cells into Smooth Muscle Cells upon Cocultivation. Tissue Eng. 2012;18:2395-2405.
- Bone marrow–derived mesenchymal stem cells facilitate engineering of long-lasting functional vasculature. Blood. 2008;111:4551-4558.
- Effect of mechanical boundary conditions on orientation of angiogenic microvessels. Cardiovasc Res. 2008;78:324-332.
- Endothelial cell lumen and vascular guidance tunnel formation requires MT1-MMP–dependent proteolysis in 3-dimensional collagen matrices. Blood. 2009;114:237-247.
- Assessing the permeability of engineered capillary networks in a 3D culture. PLoS One. 2011;6:e22086-.
- Long-term in vivo imaging of human angiogenesis: Critical role of bone marrow-derived mesenchymal stem cells for the generation of durable blood vessels. Microvasc Res. 2008;75:308-314.
- Ulex europaeus I lectin as a marker for vascular endothelium in human tissues. Lab Invest. 1982;47:60-66.
- Optimization of microCT imaging and blood vessel diameter quantitation of preclinical specimen vasculature with radiopaque polymer injection medium. PLoS One. 2011;6:e19099-.
- Angiofil®‒mediated visualization of the vascular system by microcomputed tomography: A feasibility study. Microsc Res Tech. 2008;71:551-556.
- Subcellular imaging in the live mouse. Nat Protoc. 2006;1:775-782.
- Advances in in vivo bioluminescence imaging of gene expression. Annu Rev Biomed Eng. 2002;4:235-260.
- Molecular basis for endothelial lumen formation and tubulogenesis during vasculogenesis and angiogenic sprouting. Int Rev Cell Mol Biol. 2011;288:101-.
- Synthetic biomaterials as instructive extracellular microenvironments for morphogenesis in tissue engineering. Nat Biotechnol. 2005;23:47-55.
- The relative magnitudes of endothelial force generation and matrix stiffness modulate capillary morphogenesis in vitro. Exp Cell Res. 2004;297:574-584.
- A self-assembling peptide matrix used to control stiffness and binding site density supports the formation of microvascular networks in three dimensions. Acta Biomater. 2013;9:7651-7661.
- Effects of the mechanical properties of collagen gel on the in vitro formation of microvessel networks by endothelial cells. Tissue Eng. 2007;13:1443-1453.
- Vascular endothelial growth factor and substrate mechanics regulate in vitro tubulogenesis of endothelial progenitor cells. J Cell Mol Med. 2010;14:2436-2447.
- Evaluation of Multifunctional Polysaccharide Hydrogels with Varying Stiffness for Bone Tissue Engineering. Tissue Eng. 2013;19:2452-.
- The effect of 3D hydrogel scaffold modulus on osteoblast differentiation and mineralization revealed by combinatorial screening. Biomaterials. 2010;31:5051-5062.
- Formation of human capillaries in vitro: the engineering of prevascularized matrices. Tissue Eng Part A. 2009;16:269-282.
- Cell-laden microengineered gelatin methacrylate hydrogels. Biomaterials. 2010;31:5536-5544.
- Microfabrication technology for vascularized tissue engineering. Biomed Microdevices. 2002;4:167-175.
- Rapid casting of patterned vascular networks for perfusable engineered three-dimensional tissues. Nat Mater. 2012;11:768-774.
- An overview of tissue and whole organ decellularization processes. Biomaterials. 2011;32:3233-3243.
- Immunogenicity in xenogeneic scaffold generation: Antigen removal versus decellularization. Acta Biomater. 2014;10:1806-1816.
- Cell sheet engineering: recreating tissues without biodegradable scaffolds. Biomaterials. 2005;26:6415-6422.
- Thermo‒responsive polymeric surfaces; control of attachment and detachment of cultured cells. Makromol Chem Rapid Comm. 1990;11:571-576.
- Thermo-responsive culture dishes allow the intact harvest of multilayered keratinocyte sheets without dispase by reducing temperature. Tissue Eng. 2001;7:473-480.
- Cell sheet engineering for myocardial tissue reconstruction. Biomaterials. 2003;24:2309-2316.
- Pre-vascularization of in vitro three-dimensional tissues created by cell sheet engineering. Biomaterials. 2010;31:3903-3909.
- Fabrication of functional three-dimensional tissues by stacking cell sheets in vitro. Nat Protoc. 2012;7:850-858.
- The rapid anastomosis between prevascularized networks on silk fibroin scaffolds generated in vitro with cocultures of human microvascular endothelial and osteoblast cells and the host vasculature. Biomaterials. 2010;31:6959-6967.
- Copolymer cell/scaffold constructs for bone tissue engineering: Co‒culture of low ratios of human endothelial and osteoblast‒like cells in a dynamic culture system. J Biomed Mater Res. 2013;101:1113-1120.
- The three-dimensional vascularization of growth factor-releasing hybrid scaffold of poly (ε-caprolactone)/collagen fibers and hyaluronic acid hydrogel. Biomaterials. 2011;32:8108-8117.
- Functional human vascular network generated in photocrosslinkable gelatin methacrylate hydrogels. Adv Funct Mater. 2012;22:2027-2039.
- Three-dimensional co-cultures of osteoblasts and endothelial cells in DegraPol foam: histological and high-field magnetic resonance imaging analyses of pre-engineered capillary networks in bone grafts. Tissue Eng. 2010;17:291-299.
- Discrepancies between metabolic activity and DNA content as tool to assess cell proliferation in cancer research. J Cell Mol Med. 2010;14:1003-1013.
- The proliferation and differentiation of osteoblasts in co-culture with human umbilical vein endothelial cells: an improved analysis using fluorescence-activated cell sorting. Cell Mol Biol Lett. 2010;15:517-529.
- An endothelial cell growth factor from bovine hypothalamus: identification and partial characterization. Proc Natl Acad Sci. 1979;76:5674-5678.
- Human macrovascular endothelial cells: optimization of culture conditions. In Vitro Cell Dev Biol-Anim. 2000;36:125-132.
- VEGF differentially activates STAT3 in microvascular endothelial cells. FASEB J. 2003;17:1562-1564.
- Endothelial cells from diverse tissues exhibit differences in growth and morphology. Microvasc Res. 1998;55:65-76.
- SDF-1 activity on microvascular endothelial cells: consequences on angiogenesis in in vitro and in vivo models. Thromb Res. 2000;99:587-594.
- Heterogeneity of microvascular endothelial cells isolated from human term placenta and macrovascular umbilical vein endothelial cells. Eur J Cell Biol. 2003;82:163-173.
- Concise Review: No Breakthroughs for Human Mesenchymal and Embryonic Stem Cell Culture: Conditioned Medium, Feeder Layer, or Feeder‒Free; Medium with Fetal Calf Serum, Human Serum, or Enriched Plasma; Serum‒Free, Serum Replacement Nonconditioned Medium, or Ad Hoc Formula? All That Glitters Is Not Gold!. Stem Cells. 2007;25:1603-1609.
- Alternatives to the use of fetal bovine serum: serum-free cell culture. Altex. 2003;20:275-281.
- Effect of serum on human bone marrow stromal cells: ex vivo expansion and in vivo bone formation. Transplantation. 2000;70:1780-1787.
- Optimization of the culturing conditions of human umbilical cord blood‒derived endothelial colony‒forming cells under xeno‒free conditions applying a transcriptomic approach. Genes Cells. 2010;15:671-687.
- Human umbilical cord blood plasma can replace fetal bovine serum for in vitro expansion of functional human endothelial colony-forming cells. Cytotherapy. 2011;13:712-721.
- Serum factors involved in human microvascular endothelial cell morphogenesis. J Lab Clin Med. 2002;140:188-198.
- Humanized large-scale expanded endothelial colony–forming cells function in vitro and in vivo. Blood. 2009;113:6716-6725.
- Human endothelial colony‒forming cells expanded with an improved protocol are a useful endothelial cell source for scaffold‒based tissue engineering. J Tissue Eng Regen Med. 2013.
- Microfabricated poly (ethylene glycol) templates enable rapid screening of triculture conditions for cardiac tissue engineering. J Biomed Mater Res. 2009;89:616-631.
- Biofabrication enables efficient interrogation and optimization of sequential culture of endothelial cells, fibroblasts and cardiomyocytes for formation of vascular cords in cardiac tissue engineering. Biofabrication. 2012;4:035002-.
- Vascularization strategies for tissue engineering. Tissue Eng. 2009;15:353-370.
- Oxygen regulates vascular endothelial growth factor-mediated vasculogenesis and tubulogenesis. Dev Biol. 1997;183:139-149.
- Hypoxia-inducible factor-1-dependent mechanisms of vascularization and vascular remodelling. Cardiovasc Res. 2010;86:236-242.
- Vascular endothelial growth factor induced by hypoxia may mediate hypoxia-initiated angiogenesis. Nature. 1992;359:843-845.
- The interactions between brain microvascular endothelial cells and mesenchymal stem cells under hypoxic conditions. Microvasc Res. 2008;75:59-67.
- Hypoxia promotes chondrogenesis in rat mesenchymal stem cells: A role for AKT and hypoxia‒inducible factor (HIF)‒1α. J Cell Physiol. 2008;216:708-715.
- Hypoxia inhibits the differentiation of mesenchymal stem cells into osteoblasts by activation of Notch signaling. Exp Mol Pathol. 2012;9:33-39.
- Effect of endothelial cells on bone regeneration using poly (L‒lactide‒co‒1, 5‒dioxepan‒2‒one) scaffolds. J Biomed Mater Res. 2011;96:349-357.
- Contrasting Effects of Vasculogenic Induction Upon Biaxial Bioreactor Stimulation of Mesenchymal Stem Cells and Endothelial Progenitor Cells Cocultures in Three-Dimensional Scaffolds Under In Vitro and In Vivo Paradigms for Vascularized Bone Tissue Engineering. Tissue Eng. 2012;19:893-904.
- Development of tissue engineered vascular grafts. Curr Pharm Biotechnol. 2007;8:43-50.
- Vascular endothelial growth factor molecular and biological aspects. Advances Organ Biol. 1999;7:25-57.
- Influence of adult mesenchymal stem cells on in vitro vascular formation. Tissue Eng Part A. 2009;15:1751-1761.
- Mesenchymal stem/stromal cells as a pharmacological and therapeutic approach to accelerate angiogenesis. Pharmacol Ther. 2014;143:181-196.
- Cardiac myofibroblasts: a novel source of vascular endothelial growth factor (VEGF) and its receptors Flt-1 and KDR. J Mol Cell Cardiol. 2003;35:277-286.
- Molecular mechanisms and clinical applications of angiogenesis. Nature. 2011;473:298-307.
- Requisite role of angiopoietin-1, a ligand for the TIE2 receptor, during embryonic angiogenesis. Cell. 1996;87:1171-1180.
- Angiopoietin-1 induces sprouting angiogenesis in vitro. Current Biol. 1998;8:529-532.
- Role of chemokines in angiogenesis: CXCL12/SDF‒1 and CXCR4 interaction, a key regulator of endothelial cell responses. Microcirculation. 2003;10:359-370.
- Platelet-derived growth factor indirectly stimulates angiogenesis in vitro. Am J Pathol. 1993;142:1119-.
- PDGF and vessel maturation. Angiogenesis inhibition. 2010.:103-114.
- Endothelial-mural cell signaling in vascular development and angiogenesis. Arterioscler Thromb Vasc Biol. 2009;29:630-638.